Making the First Transgenic Ants
Engineered ants are helping to reveal the neuroscience of pheromone signaling.
Taylor Hart writes about recording the neuronal activity of transgenic ants as they sense pheromones for Issue 01. Read it on our website here.
I. Seven Years
Aristotle designated humans as the highest form of “social animal.” But sociality has emerged several times throughout the course of evolution, and eusocial (“true-social”) insects, such as ants, termites, and many bees and wasps, are arguably the true exemplars.
Ants, the focus of my research, have lived in close-knit and highly cooperative family groups for more than 100 million years. They’ve evolved elaborate ways to communicate across thousands of individuals, all without relying upon a centralized hierarchy.
Whereas we use spoken and written language, ants and other social insects emit pheromones, which are odorant compounds that signal for different behaviors and allocate tasks. An ant’s pheromone repertoire is vast, and includes recruitment pheromones which signal other ants to follow a trail for group foraging and alarm pheromones which ready the colony against a threat.
As ants evolved more complex social interactions and survival strategies over time, the parts of their brain linked to olfaction, and the interpretation of these pheromones, greatly expanded. The sophistication of the ant olfactory system may offer valuable lessons for neuroscientists seeking to understand the evolution of communication in higher species, too, including ourselves.
Unfortunately, it’s difficult to study ants, let alone their brains. The neurobiology research field has grown a lot in recent decades, thanks in part to new methods to record neural activity from living organisms. Often, this is done by engineering an organism to take up a new piece of DNA, called a transgene, that is then expressed in the animal’s brain. If that transgene encodes a protein such as GCaMP, a calcium sensor that glows a soft fluorescent green each time a neuron fires, then it becomes possible to directly record brain activity as an animal encounters new stimuli, such as a taste or smell. But this technology had never worked in ants, until recently.
As a graduate student at Rockefeller University, I was part of the team that made the world’s first transgenic ants. Our results were published in the journal Cell in 2023, after seven years of precise and painstaking work. Our transgenic ants express GCaMP within their neurons, enabling us to directly visualize and record brain activity as the ants detect pheromones and odorants. Our findings also revealed a key region of the ant brain that we believe allows these insects to discern alarm pheromones and launch a collective colony defense.
This is the story of how we engineered these ants.
II. Egg Injections
When I began my Ph.D., neuroscience research on ants and other social insects was largely stagnant. The complicated, social organization that had drawn me to these animals in the first place was also the very thing that made them challenging to work with.
The queens of most social insect species will not mate or establish a new colony if they are taken out of their natural environment. It is exceedingly difficult to emulate these conditions in the laboratory, and so generating a transgenic strain of ants seemed futile.
Still, as I began my Ph.D., researchers were celebrating a big breakthrough in ant genome editing. A team led by my advisor, Daniel Kronauer, as well as another team at New York University, simultaneously reported the first CRISPR gene-edited ants. Both labs had cleverly overcome some of the experimental difficulties of ant social complexity by using species of ants with unusual reproductive strategies. The Kronauer Lab team focused on a peculiar species, called the clonal raider ant, that is capable of reproduction without a queen. They are also parthenogenetic, which means they can produce offspring without having sex. Easier to raise in the laboratory, clonal raider ants are well-suited to gene-editing experiments.
To produce these mutant ants, the researchers collected thousands of eggs, carefully poked them with a sharp, glass needle, and injected each one with a mutagenic solution containing a CRISPR DNA-cutting enzyme. CRISPR gene editing works like a pair of scissors that snip DNA at a particular site. The cell patches up the incision, but small errors accumulate and can alter a gene's function. In ants, though, the efficiency was extremely low: out of more than 3,000 injected ant eggs, just 42 reached adulthood, and only 7 mutant varieties were created. That’s a success rate of less than one percent. In other insects, such as the vinegar fly, the success rate for CRISPR gene-editing can be 35 times greater. The Kronauer Lab’s ants were mutants, but not transgenic, because no foreign DNA had been inserted into their genome.
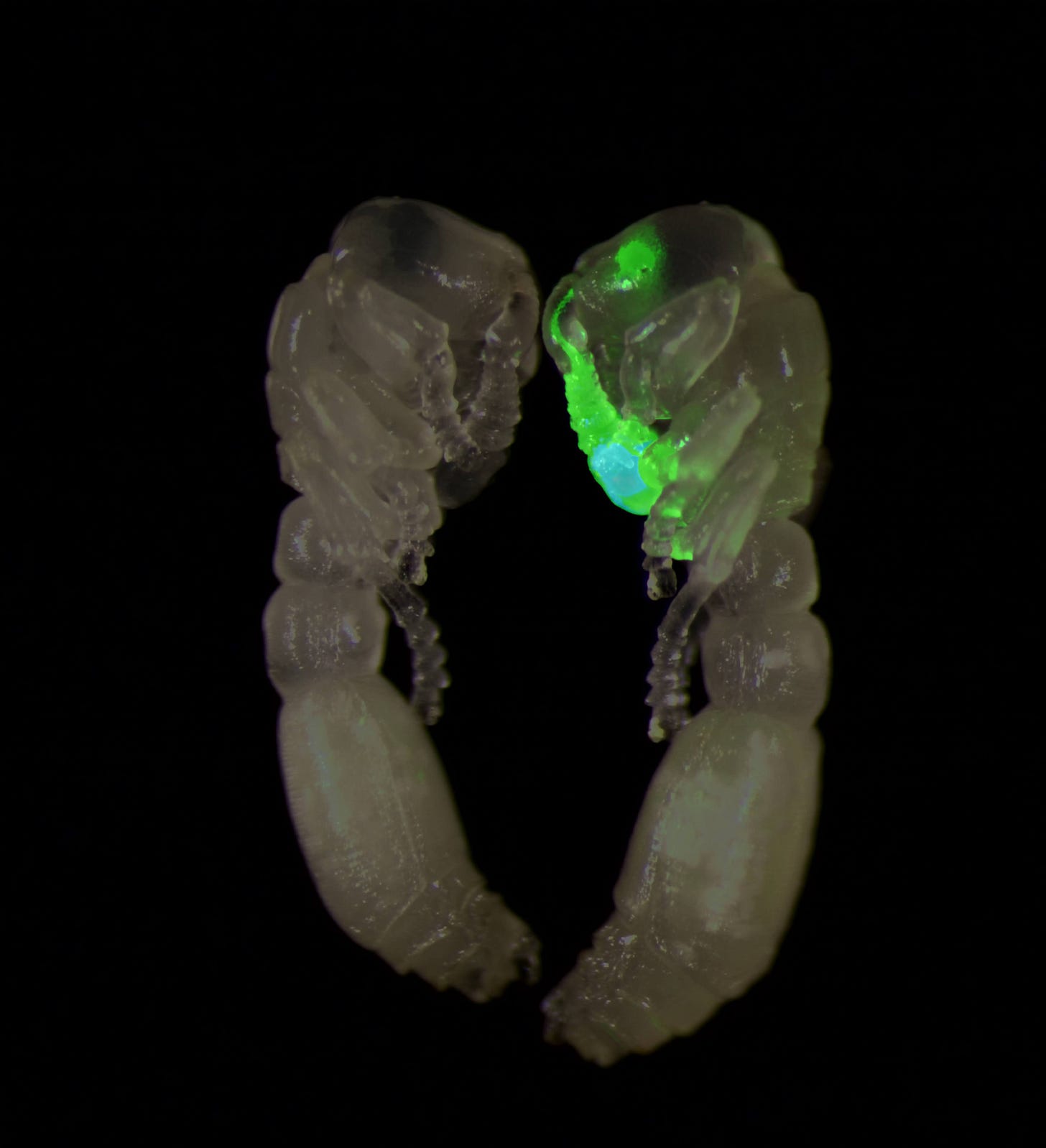
As a fresh-faced Ph.D. student, I wanted to take the leap to transgenic ants and insert a transgene encoding GCaMP into the ants’ genomes—where it would be expressed in neurons and relay feedback about what was taking place inside their brains.
There are two primary ways to accomplish that. One could inject ant eggs with the CRISPR cutting enzyme and a piece of "insert" DNA. When the CRISPR enzyme cuts the genome, the “insert” will occasionally fill in the gap, resulting in an animal that now carries a new gene that can be passed onto the next generation. But this method is about ten-times less efficient than using CRISPR just to cleave a gene because the insert needs to quickly slide in before the damage is repaired. I figured I’d have to inject about ten thousand eggs to produce a handful of transgenic survivors for my experiments, with a theoretical success rate that would probably approach 0.1 percent at best. (Toward the end of my Ph.D., my thesis committee revealed that they were initially skeptical that I’d ever manage to make it work.)
Fortunately, I learned that there’s another way to make transgenic ants using something called a transposase.
Molecular biologists knew about transposons, or “jumping genes,” long before they discovered CRISPR. Transposons are DNA sequences that can hop from one part of the genome to another. Each transposon encodes a protein that recognizes a particular stretch of DNA, normally part of the transposon itself, and inserts that sequence elsewhere in a pseudo-random manner — it can be likened to CTRL-C + CTRL-V, but for DNA.
A transposon system, called piggyBac, had previously been “hijacked” to deliver transgenic DNA into honey bees, another eusocial relative of the ants. Researchers accomplished that feat by injecting honey bee eggs with just two components: The insert, carrying piggyBac’s recognition sequences, and the transposase enzyme that physically “cuts” and “pastes” that DNA into the genome.
When these two ingredients are injected into a cell, the transposase attaches to the recognition sequences and pastes the DNA into the cell’s genome. The transposase is then degraded by the cell, while the pasted insert remains where it landed. This process has worked efficiently in injected honey bee eggs, resulting in adults that carry the inserted DNA. Based on the prior success of transposase in honey bees, and the tiny success rate of CRISPR in ants, we resolved to move forward with transposase, reasoning that it was our best shot at making a transgenic ant.
Before we could inject ant eggs, though, we needed to carefully design and build the DNA insert, using the transposon recognition sequences as a base. We wanted the DNA insert to encode a gene that, when integrated into the ant genome, would make a visible signal that could be observed with a microscope. This would make it simple to check whether our injections actually led to transgenic insects.
We initially planned to insert DNA encoding a green fluorescent protein, which would make transgenic insects glow when exposed to ultraviolet light. But the most commonly used promoters — short DNA snippets that are required for a gene to “turn on” and be expressed — are only active in the insect’s eye.1 In other words, transgenic ants with a fluorescent protein would have glowing eyes, but nothing else. But again, there was a problem: Clonal raider ants, our species of choice, do not have eyes (they are subterranean). We needed to find a fluorescent protein that could be expressed in different body tissues—ones they actually possessed.
We eventually found a promoter, taken from a virus, that had previously been used to express a fluorescent protein throughout much of the body in both flies and moths. We coupled this promoter to a gene encoding a red fluorescent protein, which would help us easily identify our transgenic ants, as if they were born with a red tag spray-painted onto them.
A DNA insert can accommodate more than just one gene, so we also elected to add the transgene encoding GCaMP. Like any gene, GCaMP needs a promoter to specify which cells should express it. Because we were compelled by the way ants communicate with one another using pheromones, I designed our construct to express GCaMP in the olfactory neurons that allow ants to smell things (using a promoter snatched from an important odorant sensing gene, called Orco). We feared that GCaMP would be expressed at too low a level for us to detect any neural activity, so we also added an additional piece of genetic machinery, called QF2/QUAS, that acts as an amplifier for genes.
If everything worked as planned, our transgenic ants would express the red fluorescent protein in some parts of their bodies and GCaMP, the calcium sensor that fluoresces when a neuron fires, in their brains’ sensory processing centers. The latter would, ideally, light up in concert with the firing of neurons, enabling us to record an ant’s brain activity, across all of its smell-sensing neurons, for the first time in history.
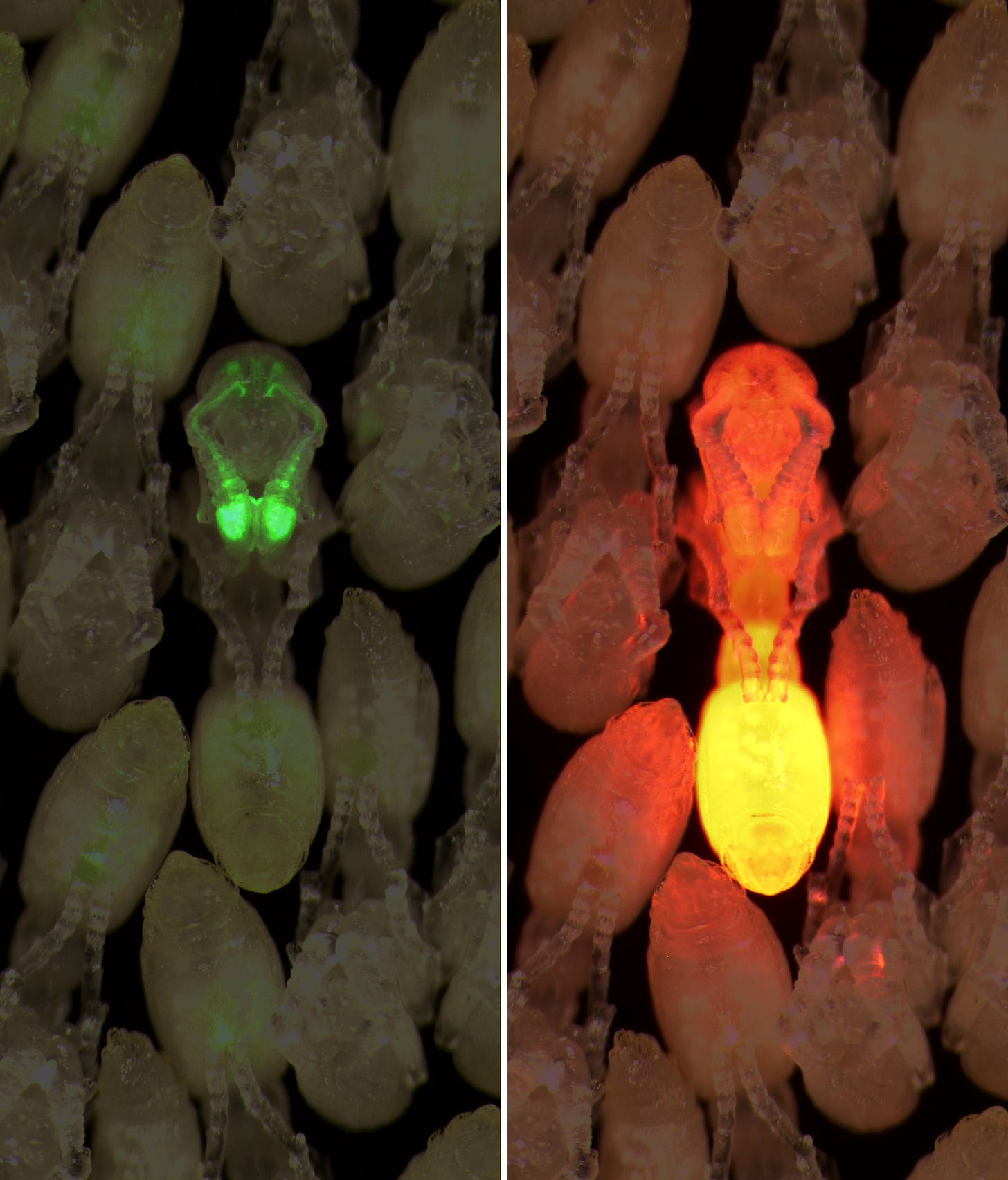
After assembling my genetic cargo, it was time to make the mutants. I borrowed heavily from the methodology for clonal raider ant egg injections that the Kronauer laboratory had previously used to make CRISPR-edited ants. The basics remained the same: Assemble a transformation mix (substituting the CRISPR components for the transposase and DNA insert), and then load it into a fine-tip, glass needle. Hold the needle with a micromanipulator rig for precise control of the needle’s 3D position using a set of dials. Connect the needle to a pressure supply, allowing the injection of fluid from the needle with the click of a computer mouse. View the needle tip and injection arena under a microscope. Carefully, break a tiny hole in the needle by tapping its tip against a piece of glass. Finally, collect freshly laid ant eggs, arrange them on a piece of tape, and inject each one with a tiny drop of the transformation mix.
I teamed up with a couple of other researchers to systematically screen dozens of small ant colonies for the freshest ant eggs. We performed this process 3-4 times per day, 3 days a week, and injected hundreds of eggs in each round.
For several months, this process yielded nothing but dead eggs. This was disheartening, but unless I wanted to scrap the experiment and my degree, I had no choice but to repeat the process again and again. After testing many different ways of injecting the eggs, I learned that my eggs were dying because I had been injecting them with too much liquid. But here and there, a handful of my injected eggs hatched into tiny larvae. I slowly figured out how much fluid went into each egg, by carefully watching the ripples that formed in the egg yolk during each injection through my microscope lens. I also learned that, at high concentrations, the transposase in my injection mix was toxic.
After adjusting my recipe accordingly and repeating the injections, I began to see small regions of red fluorescence, which were absent from the larvae that had been injected with water alone. However encouraging the results, it would take months longer before the fluorescence patterns became consistent and irrefutable.
Eighteen months into my Ph.D. research, I had mastered the ant egg injection technique, learned how to improve the survival rate of larvae, and injected more than 4,600 ant eggs. Some of the ants survived, and by examining them under a microscope, I could clearly see red fluorescence throughout the body and GCaMP expression in the sensory neurons located in the antennae and head. These ants were everything I had hoped for.
It worked.
III. Stepwise Progress
Under ideal conditions, each clonal raider ant can produce a small handful of eggs per week. Over many months of assiduous ant husbandry, I collected dozens, and eventually hundreds, of ants in this way. Now and then, I spotted ant offspring that carried the red glow of the fluorescent protein, proving that the transgene was heritable and stable. But many offspring died after a few days, presumably because the transposase had inserted our transgenes into essential sites within the genome, disrupting important genes in the ant that are required for their survival.
Eventually, I obtained hundreds of descendants of one single transgenic founder, a well-controlled set of experiment subjects with fluorescence patterns that looked bright and indistinguishable from one another.2 The GCaMP protein was expressed in about 99 percent of the antennal lobe brain region, where information from the sense of smell is processed.
Creating the transgenic ants was a long and arduous journey, but still only the precursor to implementing them in neuroscientific experiments. Through a series of collaborations with my labmates, we learned as much as we could about these mutant ants. Importantly for our experiment plans, when the transgenic ants were wafted with an alarm pheromone, they began to run about and evacuate their nest in the same ways as wild-type ants, suggesting that the added genes had not disrupted their sense of smell––the very thing we wanted to study.
In parallel, I learned to record neural activity from the ant brain by immobilizing an insect under a microscope, covering the top of its head with a saltwater and sugar solution, and then making a small hole in its exoskeleton to reveal the brain. The saltwater and sugar flood into the insect’s brain and keep it from drying out, which would otherwise happen very quickly. When the experiment is over, and the brain activity has been recorded, the ants are euthanized.
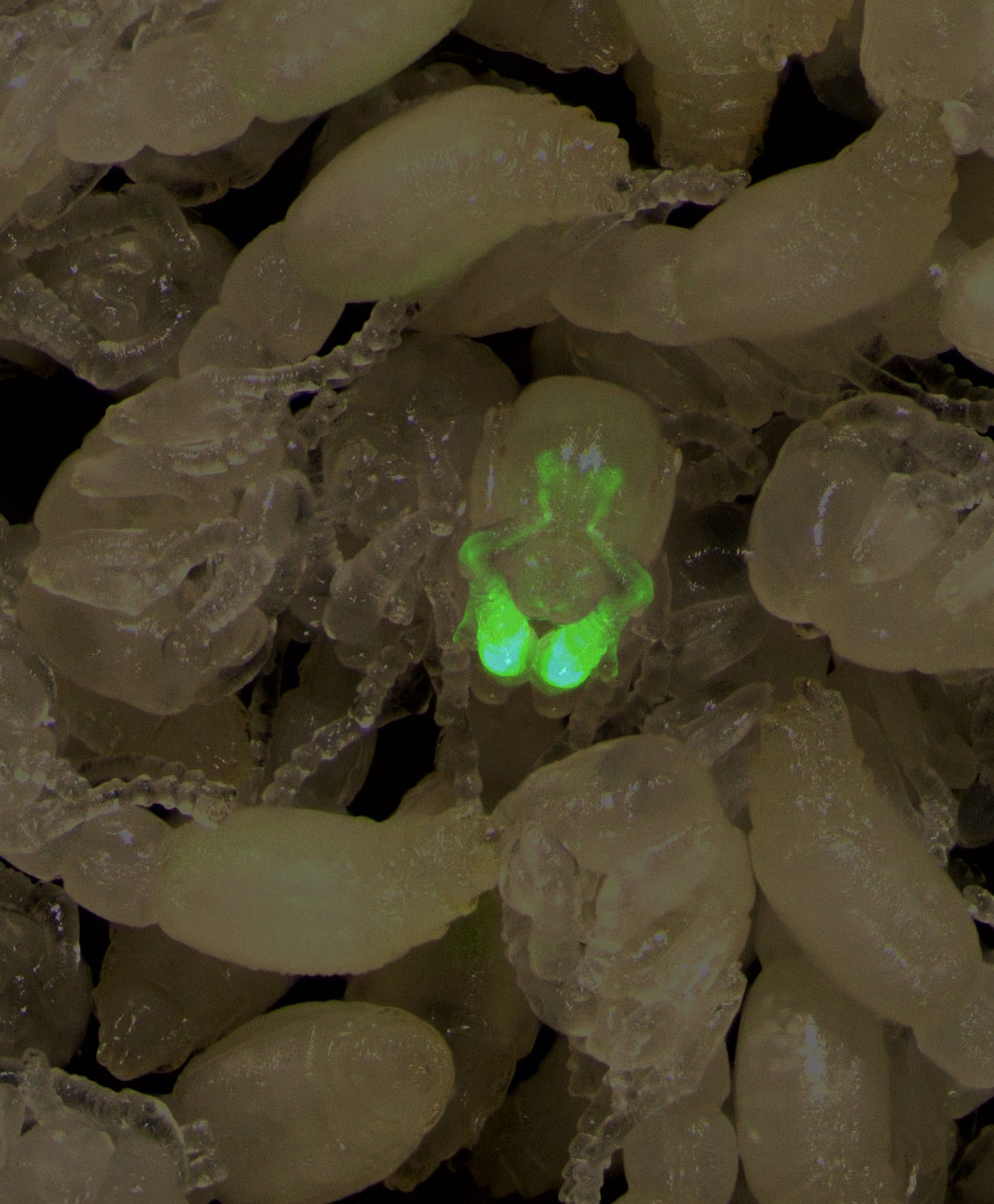
During the experiment, extreme care must be taken to avoid damaging the delicate antennae or the brain itself, which is less than half of a millimeter across. Standard methods for this procedure had been developed for more commonly studied species like the vinegar fly, but not for the clonal raider ant.3 Suffice it to say that making these methods work in our ants involved extensive trial and error. Eventually, I got to the point where I could reliably stimulate ants with a perfumed stream of air, and watch through the microscope as tiny brain areas blazed brightly in response.
After all these years of technique development and preparation, I had time left in my program to carry out one major neuroscience study. I decided to focus on the ants’ ability to communicate the presence of a threat, a project that involved mapping which parts of the ant brain respond to alarm pheromones, as opposed to other, non-pheromone odorants.
In the clonal raider ant, the main olfactory processing brain center contains more than 500 subcompartments, or glomeruli, each of which responds to odorants in different ways. But it turns out that only six of these compartments responded to the alarm compounds, suggesting that ant brains have specialized neural architectures solely for processing this kind of pheromone.
We also discovered a brain subcompartment that reliably responded to three different alarm pheromone compounds, suggesting a key role for this region in prompting the ant to launch its defensive behavior.
These findings themselves are revelatory, but more amazing still, are what they demonstrate about the progress we have made in interpreting the communication of eusocial insects.
Beyond our sociality, it was humans’ ability to communicate through language that compelled Aristotle to place us at the pinnacle of social species. Even now, centuries later, biologists have still not discovered comparable linguistic feats within the non-human world. But our ears are not attuned to the “speech” of other species, nor is it always “speech” we are evaluating. We have only learned to interpret the various aspects and sensory modalities of insect communication through intensive empirical research.
Between the 1940s and 1970s, the ethologist Karl von Frisch and his colleagues studied how bees communicate the location of food caches and new nests to other members of their colony. Von Frisch’s experiments were elegant and meticulously designed, yet could only document external communicative behavior, rather than elucidate what was actually going on inside a bee’s brain. Like ants, much of bee communication happens by way of pheromones and is therefore invisible to the eye.4 What was visible for von Frisch were the outward movements of the bee, which led him to his acclaimed discovery of the so-called “waggle dance.”
Transgenics and microscopy are changing our reliance on external behavioral proxies. We can now watch as an ant perceives a pheromone signal used in communication with a social partner, and see its neurons light up in response. Though we still have lots to learn about the nuances of this chemical, pheromonal “language”, our newfound access to the ant’s brain will doubtless aid us in understanding just how far the complexity of insect communication reaches.
Taylor Hart is a postdoctoral researcher studying how brains encode sensory information.
Cite this essay: Taylor Hart. "Making the First Transgenic Ants." Asimov Press (2024). DOI: https://doi.org/10.62211/762hd-9tp3
Footnotes
This is a purposeful choice: usually, it’s easier to see fluorescence through eye tissue compared to body parts covered in the exoskeleton.
As a coda on the ant transgenics project, I went on to generate a total of four additional transgenic lines over the next year. (In one case, I duplicated the same transgenic ant that I’ve described here, but did so after only 353 injections, rather than many thousands!) With all the experience I had built up, making each of these new lines required only a few weeks of injections, which felt like a breeze compared to the first one.
These methods have been used in a multitude of studies over 20 years, and have contributed greatly to our detailed understanding of the fly sense of smell. See here for a video overview of how calcium imaging of olfactory responses in the fly brain is done.
Wonderful piece, it would be great to hear more behind-the-scenes stories of science like this!
Incredibly impressive. It boggles my mind to think of the delicacy and precision involved in such work. Kudos to the author for pushing through to complete his PhD and bring a new strain of transgenic ants into existence!