Reservoirs of Resistance
By studying the millennia-old arms race between soil-dwelling microbes, scientists can pre-empt antibiotic resistance before it emerges in people.
On a summer day in 1924, President Calvin Coolidge’s teenage sons, Calvin Jr. and John, played lawn tennis at the White House. Calvin Jr. didn’t wear socks with his shoes and got a blister. Before long, he showed signs of infection and spiked a fever. He was rushed to Walter Reed Army Medical Center, one of the best hospitals of the day, but despite the doctors’ efforts, sixteen-year-old Calvin Jr. was dead within a week.
The microorganism that so quickly took the life of this hapless teenager was Staphylococcus aureus. Found on most people’s skin, this bacterium is relatively harmless — unless it enters the bloodstream, where it can cause fatal infection. For most of human history, bacterial infections were the leading cause of death. Today, that’s no longer the case, thanks to the discovery of antibiotics.
After sulfa antibiotics became widely available in the U.S. in the mid-1930s, for example, they led to a 36 percent decline in death rates from maternal conditions, a 24 percent decline in influenza and pneumonia death rates, and an estimated 3 percent decline in death rates overall, according to Our World in Data.
However, the paradox of antibiotics is that their very use selects for the emergence and spread of antibiotic resistance: bacteria evolve mechanisms to survive the drugs that once killed them.1 In the United States, more than 2.8 million antibiotic-resistant infections occur each year, with more than 35,000 patients dying as a result. Experts have speculated that we are careening toward a “post-antibiotic” era, where “routine surgery or chemotherapy is considered too dangerous because there are no drugs to prevent or treat bacterial infections.”2
Historically, scientists and clinicians have studied the genes that cause antibiotic resistance only after they’re found in disease-causing pathogens. But in recent decades, microbiologists have discovered that environmental bacteria — particularly soil-dwelling microbes — encode for resistance genes that are vastly more diverse than those found in human pathogens. They have also found that many of the same resistance genes employed by multidrug-resistant pathogens originally evolved in soil-dwelling bacteria millennia ago, and only jumped into pathogens recently. Studying the well-stocked reservoirs of resistance in soils could therefore help us identify and develop countermeasures against emerging resistance threats before they spread in people.
The origins of antibiotic resistance are intimately connected with those of antibiotic production. Most of our clinically important antibiotics come from microbes, particularly a group of soil-dwelling bacteria called the actinomycetes.3 Precisely dating the origins of antibiotic-producing bacteria is difficult, but estimates range from 40 million to 2 billion years ago.
In 1973, Julian Davies and colleagues at the University of Wisconsin—Madison hypothesized that the age of antibiotic resistance is likely just as old as antibiotic production because microbes that make antibiotics must also have mechanisms to protect themselves against those same antibiotics. Otherwise, they’d be susceptible to their own product.
This “producer hypothesis” stemmed from Davies’ observations that various species of soil-dwelling Streptomyces, a genus famous for producing aminoglycoside antibiotics such as streptomycin and gentamicin (which work by binding to the bacterial ribosome, interrupting protein synthesis), also encode for enzymes that inactivate aminoglycosides in a manner similar to resistance enzymes produced by pathogens.
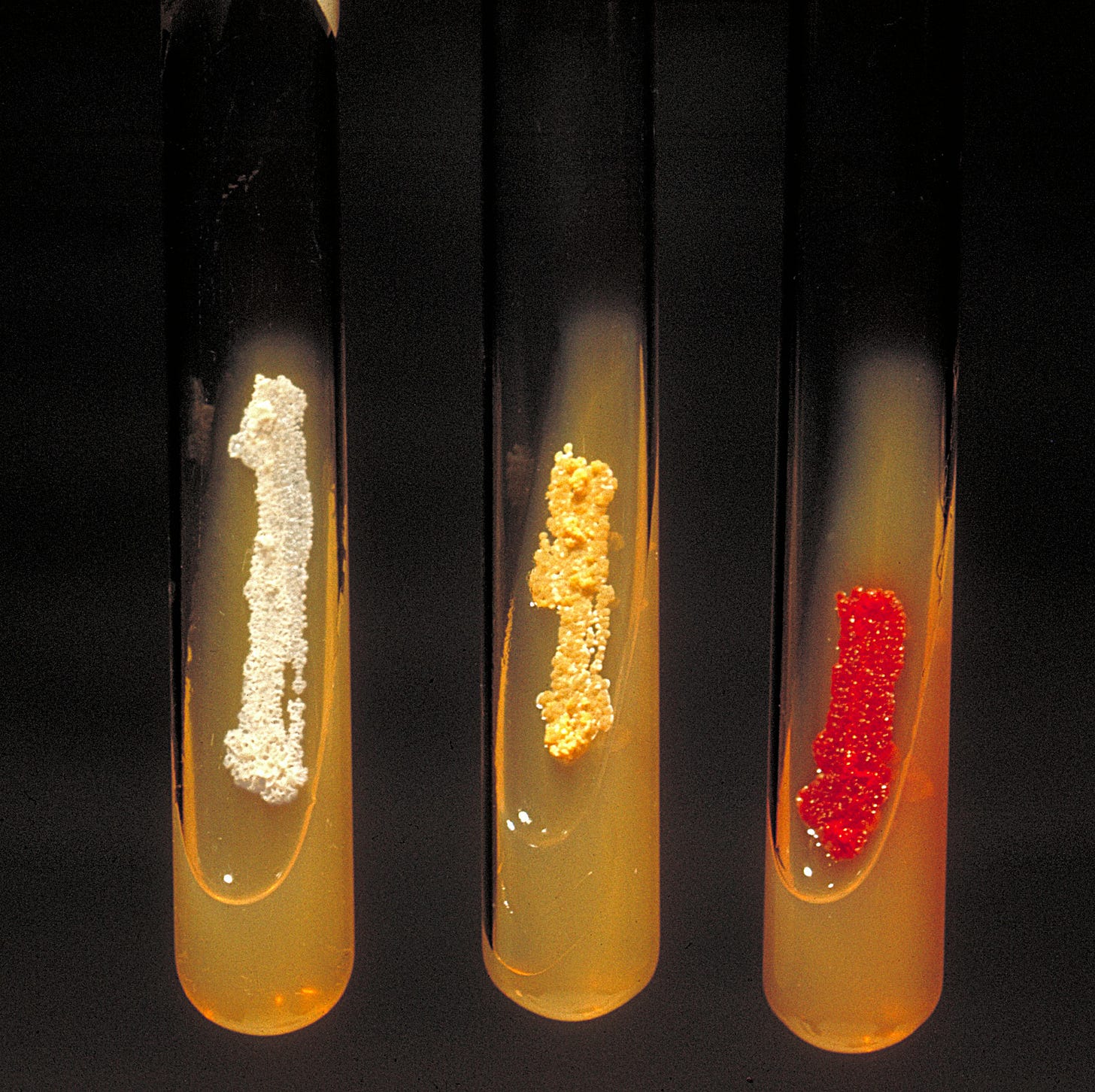
Microbes that don’t make antibiotics may have acquired antibiotic resistance genes from the microbes that do via horizontal gene transfer. Resistance genes get packaged into mobile elements, such as plasmids, and swapped between different bacterial cells, including between species separated by great evolutionary distances. This allows pathogens to skip the painstakingly slow process of natural selection and directly acquire pre-existing resistance genes that other species evolved over millions of years.
If antibiotic resistance originates in soil-dwelling antibiotic producers, then three predictions logically follow: soil is a potent reservoir of resistance genes, resistance in soil long predates antibiotic manufacturing by humans, and human pathogens may have acquired antibiotic resistance genes from soil-dwelling bacteria. Perhaps by answering these questions, scientists could figure out how to slow the spread of antibiotic resistance. And, fortunately, in the 50 years since Davies first advanced his hypothesis, growing scientific evidence has supported each prediction.
First, are soil bacteria resistant to antibiotics? In 2006, a landmark study by biochemist Gerry Wright and colleagues at McMaster University showed that nearly 500 randomly isolated soil-dwelling Streptomyces were, on average, resistant to 7 or 8 antibiotics. These levels of resistance exceeded most clinical pathogens. Two soil strains were resistant to 15 of the 21 drugs tested, including compounds that were entirely synthetic and recently approved for clinical use. Other censuses of cultured soil bacteria have confirmed these high levels of resistance.
Wright’s paper also introduced the concept of the “resistome,” referring to the complete collection of antibiotic resistance genes within a specific microbial community or ecosystem. However, Wright’s approach had an important limitation: it relied on isolating individual bacterial strains and growing them in the lab, which misses resistance genes carried by the vast majority of bacteria that don't grow under standard laboratory conditions.4
To overcome this limitation, researchers have developed DNA-based techniques that can detect antibiotic resistance genes directly from environmental samples. These methods often work by extracting all the genetic material from a soil sample, for example, and then using polymerase chain reaction (PCR) and DNA sequencing to identify specific antibiotic resistance genes within it, rather than trying to culture living cells.
In 2010, David Graham and colleagues at Newcastle University used this method to quantify the presence and abundance of antibiotic resistance genes within archived soil samples collected in The Netherlands. The soil samples were collected over a period spanning the start of human antibiotic production to the present day (1940 to 2008). Over the 70-year study period, Graham’s team found an increase in the levels of key beta-lactam, macrolide, and tetracycline resistance genes. Some individual antibiotic resistance genes were 15 times more abundant in 2008 than they were in the 1970s.
Although Graham’s research indicated that human production of antibiotics also increased antibiotic resistance in soils, it didn’t answer whether the resistance genes in soil predate human antibiotics. Answering this second prediction would require an approach more akin to archaeology than clinical microbiology.
Like archaeologists who study artifacts preserved over thousands of years, microbiologists studying ancient resistance genes must rely on materials that survive over long periods. DNA is particularly durable, with a half-life measured in thousands of years — far outlasting bacterial cells. Scientists can therefore study ancient resistomes by extracting preserved DNA directly from soil samples.
In 2011, Wright’s group did exactly this to analyze 30,000-year-old permafrost from the Yukon. Intermixed with DNA from wooly mammoths and bison, they found the evolutionary relatives of resistance genes against important modern antibiotics, including beta-lactams, tetracyclines, and vancomycin.5
By inserting these vancomycin resistance genes into lab bacteria, Wright’s team was also able to purify the enzymes they encoded and show that these ancient enzymes catalyze biochemical reactions consistent with modern vancomycin resistance proteins. This confirmed that these ancient genes were capable of genuine antibiotic resistance.
DNA-based studies quantify the presence or absence of antibiotic resistance genes already known to science, typically because they’ve gotten into a human pathogen. However, it is possible that this is just the tip of the iceberg. What unknown resistance genes might be lurking within the majority of bacteria we have yet to study?
By their definition, unculturable organisms can’t be studied the traditional way — by isolating individual strains and growing them on antibiotics. But Jo Handelsman, now at the University of Wisconsin-Madison, and colleagues developed an ingenious workaround using a technique called culture-independent functional metagenomics. This method inserts DNA fragments extracted from a sample (in this case, Alaskan soil) into susceptible strains of E. coli. Since these E. coli are culturable, they can be studied in the typical fashion. Any bacteria that take up the DNA fragments and become resistant to antibiotics must have an antibiotic resistance gene included within their fragment.6
Using this method, Handelsman found many different beta-lactamases, or enzymes that bacteria use to break down antibiotics, including one unique version named β-lactamase LRA-13. This enzyme is unusual because it's actually two full beta-lactamases fused together, each with its own function. Having both enzymes combined into one might help bacteria survive better, since the fused version can break down a wider variety of antibiotics. Crucially, all the beta-lactamases found in this study gave E. coli the ability to resist antibiotics at clinically-relevant concentrations. If these genes were to spread into disease-causing bacteria, then they could undermine our ability to treat infections.
The third prediction, that human pathogens acquired antibiotic resistance genes from soil-dwelling bacteria, was confirmed by a pair of studies by Gautam Dantas and colleagues at Washington University in St. Louis, though with a caveat.
In 2012, Dantas’ team used functional metagenomics to characterize the resistomes of 11 U.S. soil samples. Interestingly, half of the antibiotic resistance genes identified within were previously unknown, highlighting the vast diversity of soil resistance we know nothing about. Even more striking was the discovery that the DNA sequences of seven resistance genes from soil bacteria were 100 percent identical to genes previously sequenced in clinical isolates. Identical, full-length genes between such evolutionarily distant species could only occur as a result of recent horizontal gene transfer.
Fortunately, a follow-up study indicated that horizontal transfer of resistance genes among soil bacteria is a relatively rare occurrence. Though pathogenic species regularly swap resistance genes, antibiotic resistance genes in soil may not transfer as readily. We’re lucky that the massive reservoir of cryptic resistance genes in the soil resistome cannot flow freely into human pathogens. However, even the trickle that has occurred has been enough to threaten the gains of modern pharmaceuticals.
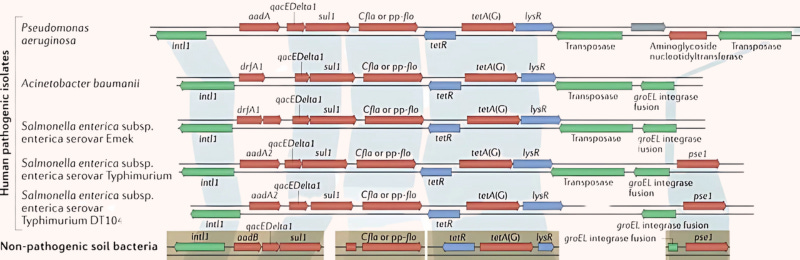
Antibiotic resistance research over the last 20 years has shown that the soil resistome is diverse, ancient, and transferable into human pathogens. And while it may be disheartening to realize that the very ground beneath our feet houses a massive and largely mysterious reservoir of antibiotic resistance, this work has also provided actionable insights for managing resistance threats.
First, recognizing that bacteria have been making antibiotics for hundreds of millions of years means it’s unlikely we’ve discovered all the potentially useful compounds that have evolved over such a long time. Ample opportunity remains to discover clinically potent compounds. Just this year, Wright’s group discovered a new class of lasso-shaped antibiotic made by slow-growing bacteria isolated from, of all places, a research technician’s garden.
Second, monitoring resistance genes in soils and other environmental reservoirs could help us prepare for future threats. DNA-based methods could provide us with an early warning system that detects the presence and abundance of resistance genes, informing where and when specific antibiotics should be used. Additionally, scientists could use functional metagenomics to screen new antibiotics against environmental resistomes, helping them identify undiscovered resistance mechanisms that might threaten the future effectiveness of these drugs.
The story of the tetracycline destructases, the subject of my doctoral research in Gautam Dantas’ lab, bears out the value of such a proactive approach. By chance, these antibiotic-inactivating enzymes were first discovered in a harmless gut bacterium. Related enzymes were also found as part of the aforementioned soil functional metagenomics studies. Though active against all generations of tetracycline antibiotics, including new derivatives approved in 2018, they were regarded as clinically unimportant because they weren’t found in human pathogens for many years. We studied them anyway, learning how they worked and developing inhibitors that block their antibiotic-degrading activity. When it was reported in 2019 that destructases had spread into several multidrug-resistant pathogens, we already had tools at hand to mitigate their spread.
The field of microbiology has historically viewed antibiotic resistance through a clinical lens, mobilizing to respond only after resistance has spread into human pathogens. While Calvin Jr.'s fatal Staphylococcus aureus infection could have been cured with penicillin, the treatment window was short: the first penicillin-resistant Staphylococcus aureus was discovered just 2 years after penicillin’s introduction. Today, there are strains of S. aureus resistant to all beta-lactam antibiotics.
Taking the long view has enabled a much-needed shift in focus. We cannot stop the evolution of antibiotic resistance — indeed, the key insight from these studies is that resistance to any new antibiotic has likely already existed for millions of years. But by understanding resistance’s ancient origins and modern spread outside the clinic, we can intervene in this process and mitigate its worst impacts. This proactive approach may be what’s needed to finally break the cycle of resistance and avoid returning to a time when even a blister could kill.
Kevin Blake is a scientific editor at Washington University in the Division of Laboratory and Genomic Medicine. He writes about microbiology, bioinformatics, and evolution.
Cite: Blake K. “Reservoirs of Resistance.” Asimov Press (2025). https://doi.org/10.62211/83fg-22rw
Lead image by Ella Watkins-Dulaney.
Alexander Fleming himself warned against antibiotic resistance in his Nobel Prize lecture: “It is not difficult to make microbes resistant to penicillin in the laboratory by exposing them to concentrations not sufficient to kill them... The time may come when penicillin can be bought by anyone in the shops. Then there is the danger that the ignorant man may easily underdose himself and by exposing his microbes to non-lethal quantities of the drug make them resistant.”
There have been no new classes of broad-spectrum antibiotics brought to market since the 1980s, according to Wellcome Trust.
Selman Waksman discovered streptomycin — most famously used to treat tuberculosis — in a strain of Streptomyces griseus; Benjamin Minge Duggar and Yellapragada Subbarao discovered tetracycline from a strain of Streptomyces aureofaciens.
Most microbial species cannot be grown in the lab due to specific nutrient requirements, environmental dependencies, or other factors.
The ancient human microbiome is also a reservoir of resistance. Antibiotic resistance genes have been found in the gut microbiome of a pre-Columbian Andean mummy from Cuzco, Peru (980-1170 CE), and from the oral microbiomes of human skeletons in a medieval monastery (950-1200 CE).
Functional metagenomics is limited in that it will miss resistance genes which are nonfunctional in the chosen host organism (e.g., E. coli).
It would be interesting to know if the most dangerous AB resistant bacteria could be treated with viral bacteriophages.
Does this imply that completely artificially derived antibiotics should be robust against antibiotic resistance?