A Brief History of GFP
How a chance discovery in bioluminescent jellyfish led to one of the most transformative tools in modern biology: green fluorescent protein.
In the summer of 1961, Osamu Shimomura sat alone in a rowboat on the Puget Sound, surrounded by thousands of glowing jellyfish. A marine biologist and chemist, he often sought out such quiet moments to reflect on his experimental failures. For two weeks, he had been struggling to isolate the light-emitting molecule, luciferin, which scientists believed was responsible for the jellyfish’s luminescence. There on the water, a new idea struck him: What if luciferin wasn’t the source of the glow at all?
This insight led to the discovery of green fluorescent protein, or GFP. While initially overlooked, GFP later revolutionized biology and medicine, earning Shimomura a share of the 2008 Nobel Prize in Chemistry. Scientists realized that GFP, a single-gene protein, functioned like a built-in cellular flashlight. By attaching the GFP gene to another gene of interest, they could track protein movements, monitor gene expression, and observe cellular processes in real time.
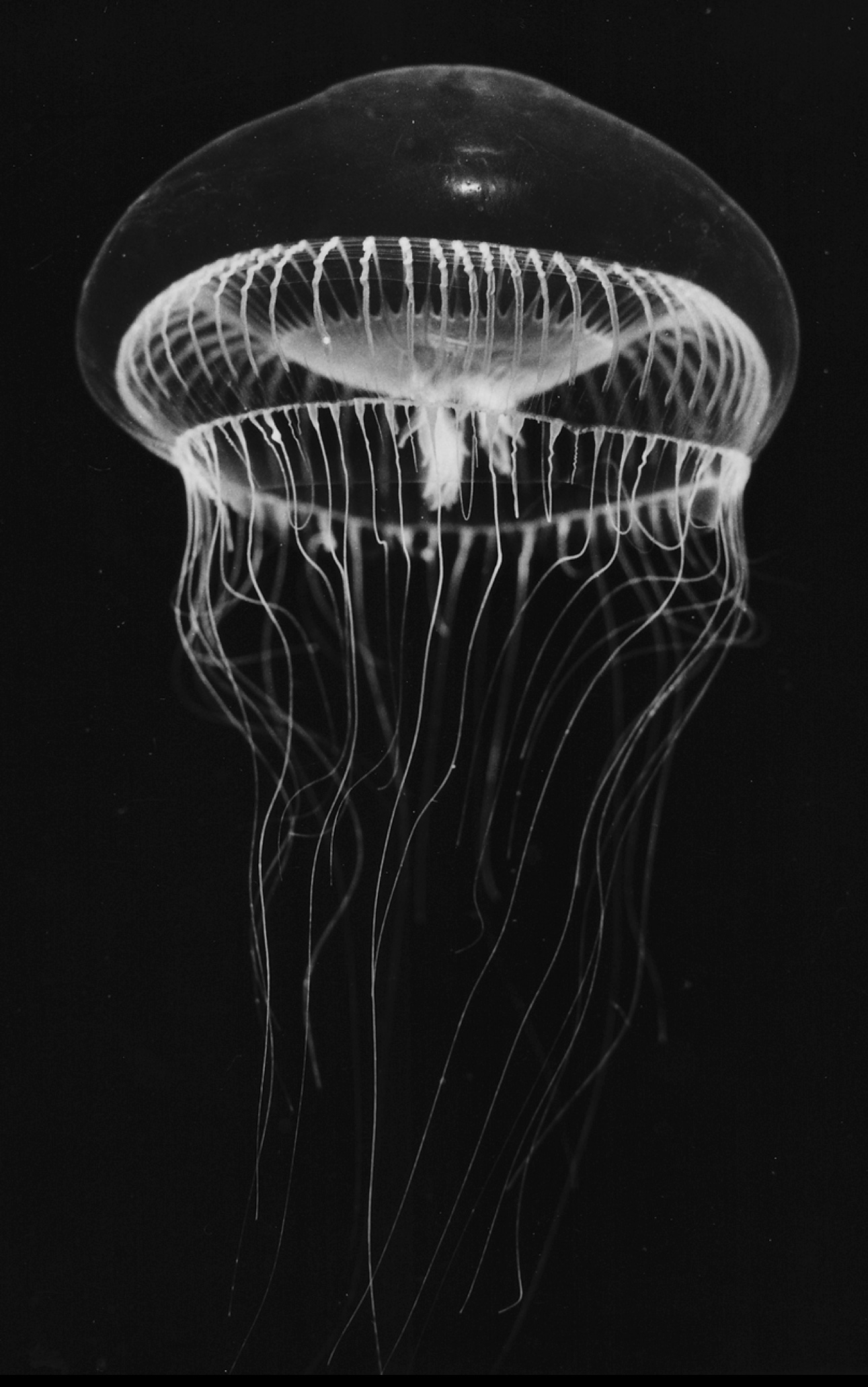
In 1999, researchers used GFP to trace the spread of cancer in a living mouse, watching tumors grow and metastasize under a fluorescence microscope. A year later, two independent teams engineered GFP into synthetic biological circuits, enabling them to watch living cells act as tiny biochemical oscillators — the start of synthetic biology. Today, scientists often visualize brain activity with modified versions of GFP, measuring when neurons fire.
GFP’s journey from an obscure footnote to a scientific cornerstone unfolded mainly through the work of four scientists. The first was Osamu Shimomura, who moved from wartime Japan to Princeton, New Jersey, to research the bioluminescent jellyfish, Aequorea victoria. He identified GFP unexpectedly as a byproduct of his work. The second, Douglas Prasher, was a biochemist at Woods Hole Oceanographic Institution in Massachusetts and the first to clone and sequence the gfp gene, in 1992. Martin Chalfie, a neuroscientist, was the first to recognize GFP’s potential as a genetic marker and demonstrate its use in living organisms. And fourth, Roger Tsien, a neurobiologist and chemist, expanded GFP’s utility by engineering a spectrum of fluorescent proteins, allowing the tracking of multiple genes and proteins simultaneously. These fluorescent proteins are now found on the benches and in the freezers of virtually every biology lab.
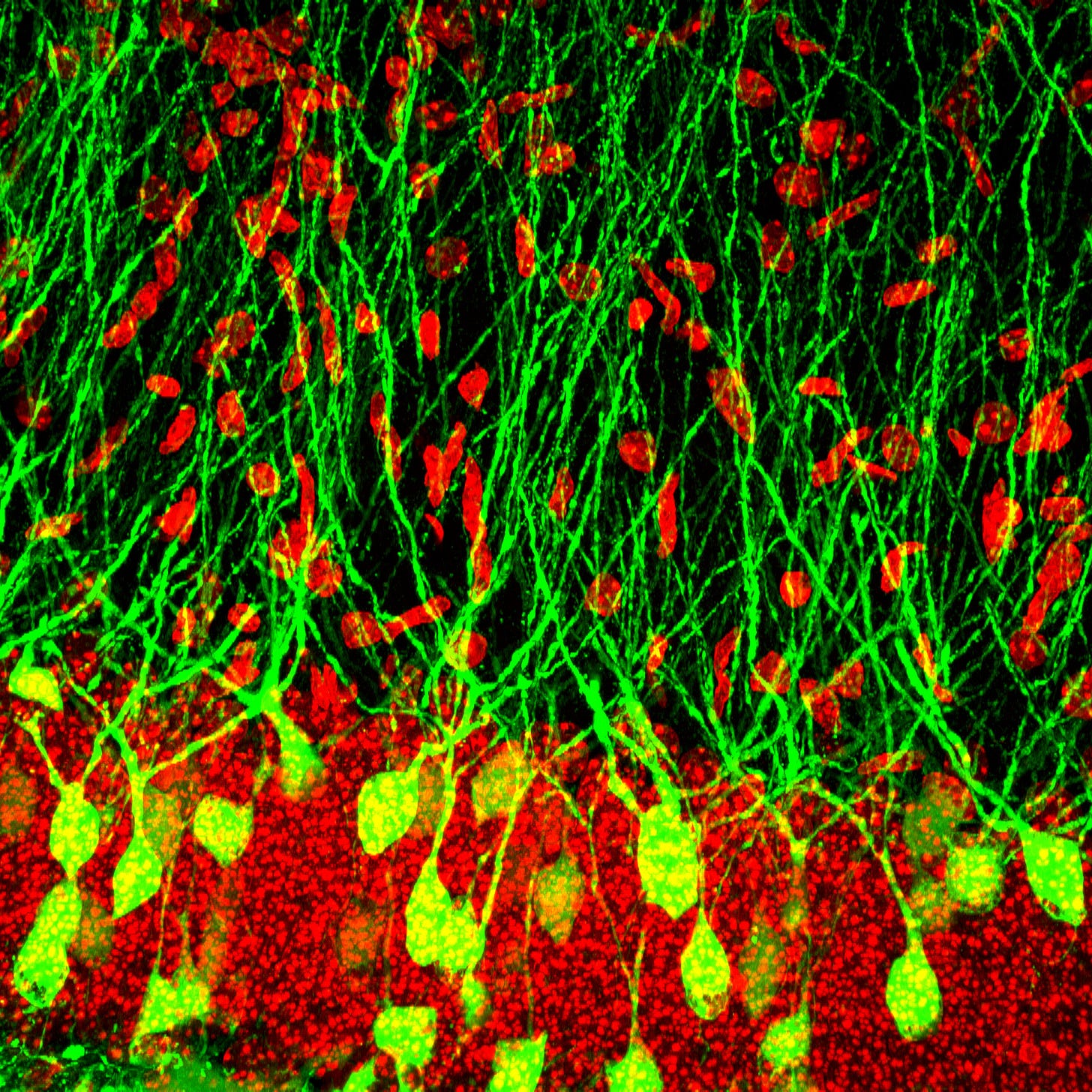
From Fukuchiyama to Friday Harbor
Osamu Shimomura was born in 1928 in Fukuchiyama, Japan. His family moved five times due to his father’s army career before settling in Isahaya, 15 miles from Nagasaki. In 1944, during his first day of tenth grade, the military mobilized his class and stationed Shimomura at a local naval aircraft factory.
On August 9, 1945, Shimomura stood atop a nearby hill and watched a B-29 bomber fly toward Nagasaki. Minutes later, Shimomura returned to his workstation just as an intense flash of light flooded the factory and blinded him for 30 seconds. That day’s devastation shaped the rest of his life, but two small twists of fate spared him from more severe consequences.
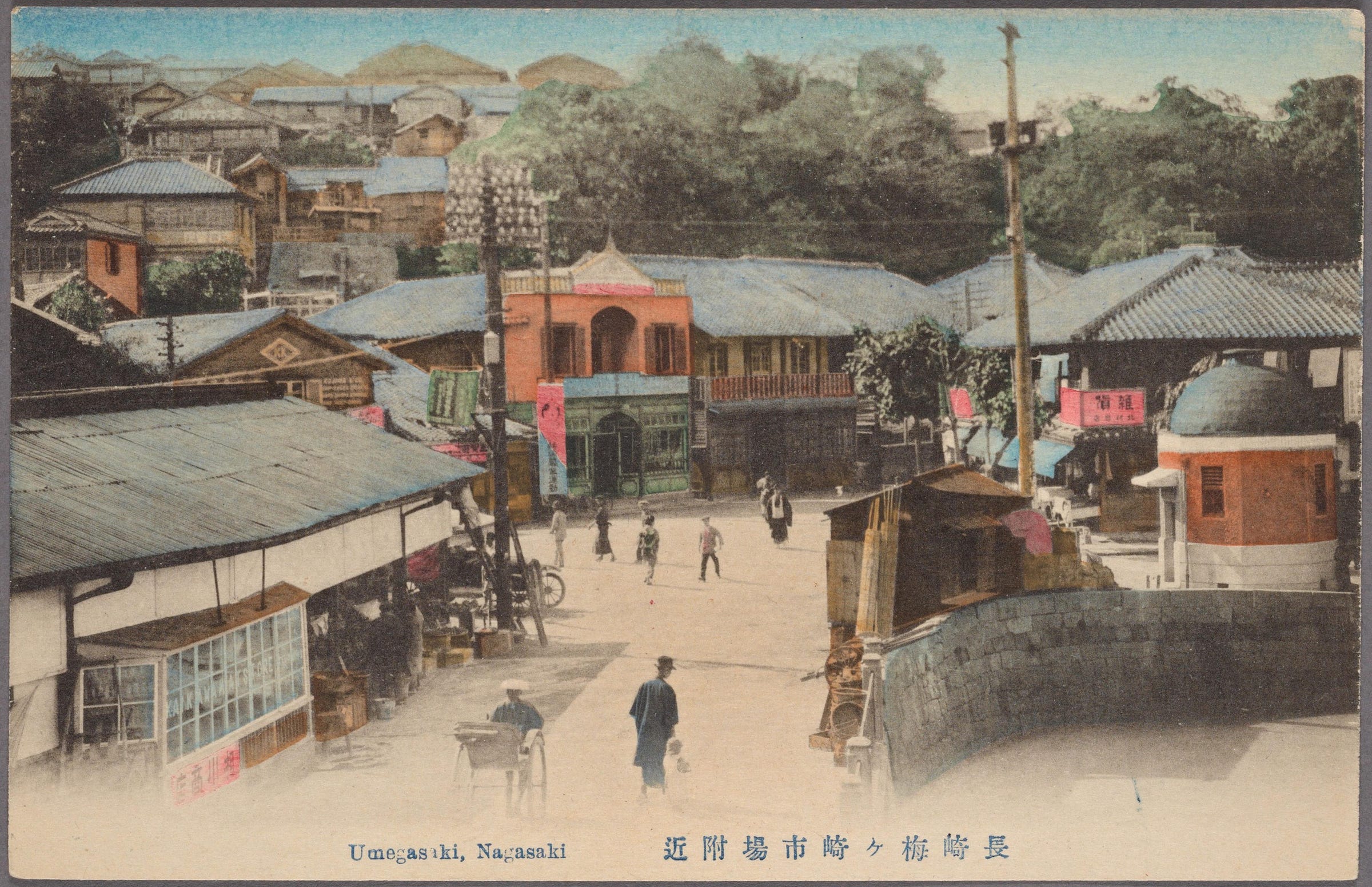
If he had stayed on the hill and continued watching as a second B-29 dropped the atomic bomb, he might have suffered permanent eye damage. And if his grandmother hadn’t insisted he bathe after returning home covered in black ash, he might have suffered severe radiation poisoning. Shimomura later reflected that such moments of fortune followed him throughout his career.
After the war, Shimomura struggled to continue his education. High schools rejected him due to low grades, and he spent two years without clear prospects. Eventually, he gained admission to Nagasaki Pharmacy College, which had been relocated to Isahaya after the atomic bombing destroyed its original site. Shimomura spent countless hours studying metallic ions and refining his skills in column chromatography.
After graduating, Shimomura remained at Nagasaki Pharmacy College as a teaching assistant before moving to Nagoya University as a research assistant under Yoshimasa Hirata. His task was to purify, crystallize, and then solve the structure of Cypridina luciferin, a light-emitting molecule from the sea firefly, a tiny crustacean (about the size of a sesame seed) common to the coast of Japan. Because the project carried a high risk of failure, it was considered unsuitable for a degree-seeking student. As Shimomura wasn’t pursuing a degree at the time, the experiments fell to him.
This project introduced him to bioluminescence. Scientists knew that the sea firefly’s luminescence resulted from a chemical reaction between luciferin and an enzyme called luciferase. But after two decades of effort, nobody had successfully crystallized luciferin, a crucial step toward identifying its structure. Shimomura spent months extracting and purifying the compound, but each attempt at crystallization failed.
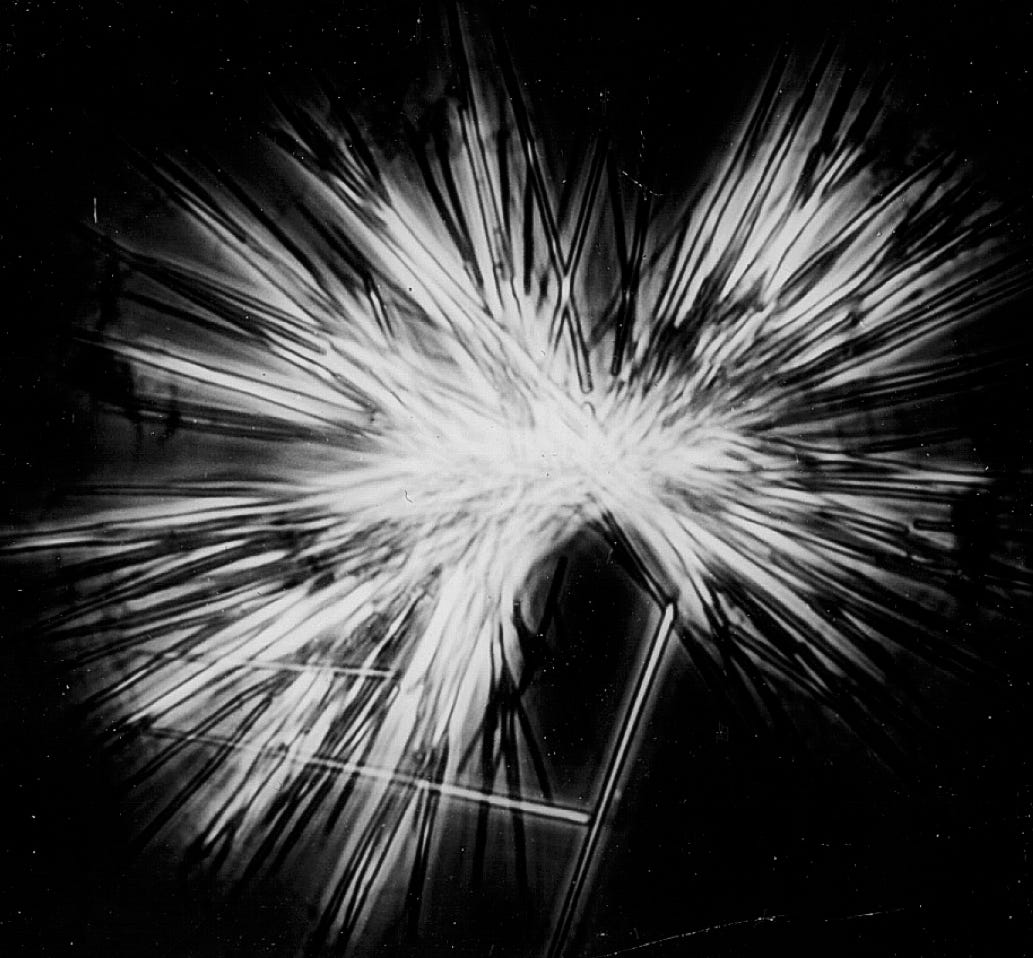
One cold February evening, after yet another unsuccessful attempt, Shimomura turned off the heat in the lab and left for the night. When he returned the next morning, he found that the dark red liquid he had left behind was now colorless and contained a crystalline precipitate. Under the microscope, this precipitate resolved into dark red needle-shaped crystals — the long-sought luciferin. The overnight drop in temperature had triggered crystallization. This breakthrough, after ten months of effort, marked Shimomura’s first major scientific success.
More good news soon followed. First, Hirata was so impressed with his young student that he granted him a PhD in organic chemistry in 1960. Shimomura’s sea firefly paper then attracted the attention of Frank Johnson, a professor of biology at Princeton University, who invited Shimomura to study with him in New Jersey. Shimomura accepted the offer and, in August 1960, departed for the United States as a Fulbright Scholar.
Upon arriving in Princeton, Johnson handed Shimomura a vial of freeze-dried jellyfish light organs and encouraged him to study their bioluminescent properties. The prevailing hypothesis at the time was that Aequorea jellyfish produced light through luciferin, a small molecule sensitive to oxygen and moisture. Johnson wanted Shimomura to purify, crystallize, and determine its properties as he had done with Cypridina luciferin. Shimomura agreed.
The following summer, Shimomura traveled to Friday Harbor, Washington, to begin collecting jellyfish.
850,000 Jellyfish
Aequorea jellyfish appear nearly transparent, with faint lines radiating outward like the ribs of an umbrella. Each jellyfish measures three inches across and weighs about 50 grams. When stimulated by touch, they emit a green glow along their outer ring, where the light organs reside. Aequorea flourished in the Puget Sound in the mid-20th century, especially in the areas around San Juan Island, where Friday Harbor is located.
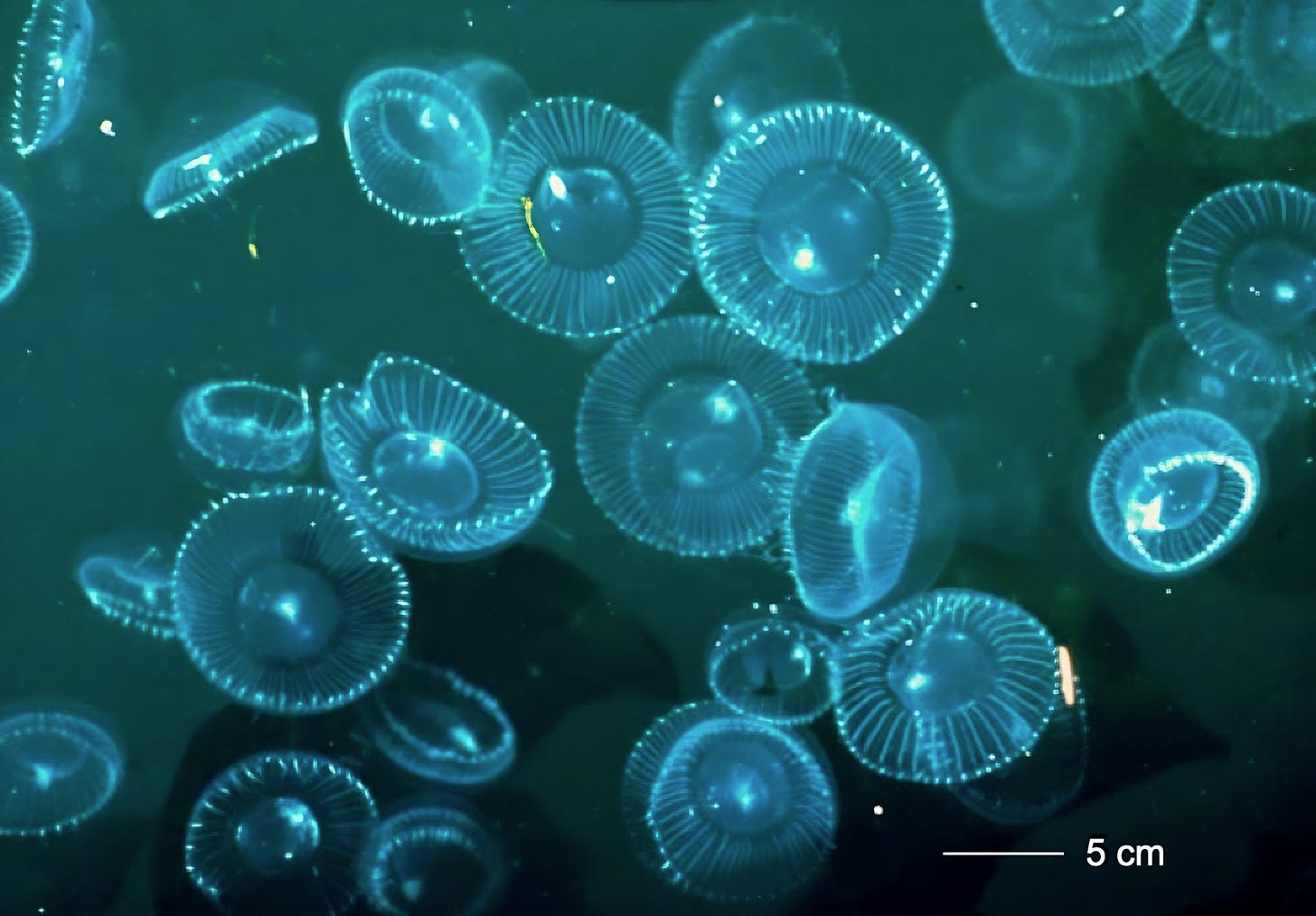
Shimomura and his wife, alongside Johnson and a research assistant, used shallow pool nets to scoop jellyfish from the harbor. Every morning and evening, they collected hundreds of specimens in large buckets. During the afternoons, they snipped two-to-three millimeter thick strips from each jellyfish’s outer ring and squeezed them through gauze to extract the luminescent substance.
Bioluminescence refers, simply, to the ability of living creatures to conjure their own light through precisely orchestrated chemical reactions. In Cypridina, for example, luciferin reacts with oxygen in the presence of luciferase to produce blue light. In Aequorea, the familiar green glow instead results from a combination of bioluminescence and fluorescence — a process in which shorter-wavelength light (blue) is absorbed and re-emitted as longer-wavelength light (green). In 1961, Shimomura was focused solely on bioluminescence, entirely unaware that the jellyfish’s glow involved an additional, unique fluorescence mechanism.
For weeks, he tried unsuccessfully to extract luciferin from the jellyfishes’ light organs. He began taking solitary boat trips on Puget Sound to ponder the failure of the luciferin-luciferase hypothesis. Then the idea came to him: “Even if luciferin and luciferase are not involved in the jellyfish bioluminescence,” Shimomura recounted in his autobiography, Luminous Pursuit, “some protein is probably involved in the bioluminescence reaction. If so, the activity of that protein would very likely be altered or affected by a change of pH.”
Shimomura began testing how acidity affects the jellyfishes’ glow by soaking them in buffers of varying pH. He found that the bioluminescent material glowed at pH 5, 6, and 7, but not at pH 4. Apparently, very acidic conditions could halt the bioluminescent reaction. And since aequorin breaks down once it emits light, Shimomura realized he needed a way to keep it intact before it “used itself up.” Bathing the jellyfish rings in an acidic buffer could stop the reaction, preserving the aequorin for further study.
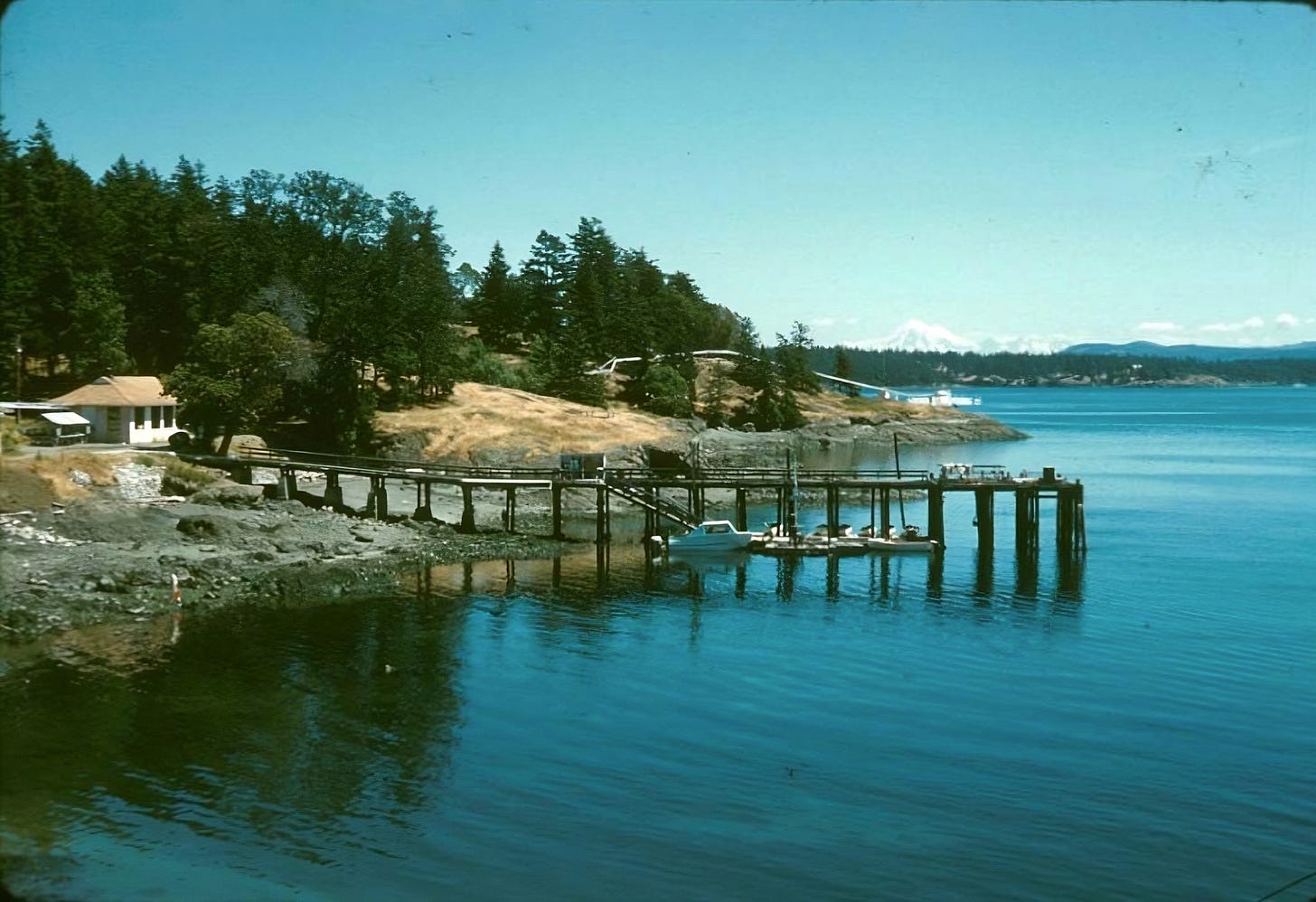
Then, as Shimomura discarded the luminescent substance down the sink, he was startled to see it glow bright blue. He knew the sink carried overflow from a salt-water aquarium and immediately suspected something in the seawater had sparked the reaction. Because there were no other plausible triggers, he hypothesized that calcium ions in the salt water caused the unexpected glow. To confirm this, he added calcium to the luminescent extract in a controlled setting and again saw the same brilliant flash of light. This finding proved that Aequorea’s bioluminescence depends on calcium. Shimomura named the light-producing protein “aequorin.”
With this new understanding, the team set out to harvest as many jellyfish as possible before the season ended. Collecting them was fairly easy, but cutting out the three-millimeter rings from slippery animals proved tedious. By the end of that first summer, they had extracted material from about 10,000 jellyfish and took it back to Princeton for further study.
Back in New Jersey, Shimomura discovered a second, green-colored protein in addition to the aequorin while running column chromatography experiments to separate out each biochemical component. He dubbed it “green protein” and linked it to the jellyfish’s natural green glow. It was renamed “green fluorescent protein” when scientists realized the color resulted from fluorescence. In a 1962 paper, Shimomura described his discovery of aequorin in great detail, but only mentioned the “green protein” in a footnote.
Aequorin’s glow in the presence of calcium was quickly harnessed for biology research. In the 1960s, many scientists suspected that calcium ions played a critical role in living organisms, but they lacked definitive evidence. In 1967, scientists at the University of Oregon used aequorin to prove that calcium ions linked nerve cell firing to muscle contractions.
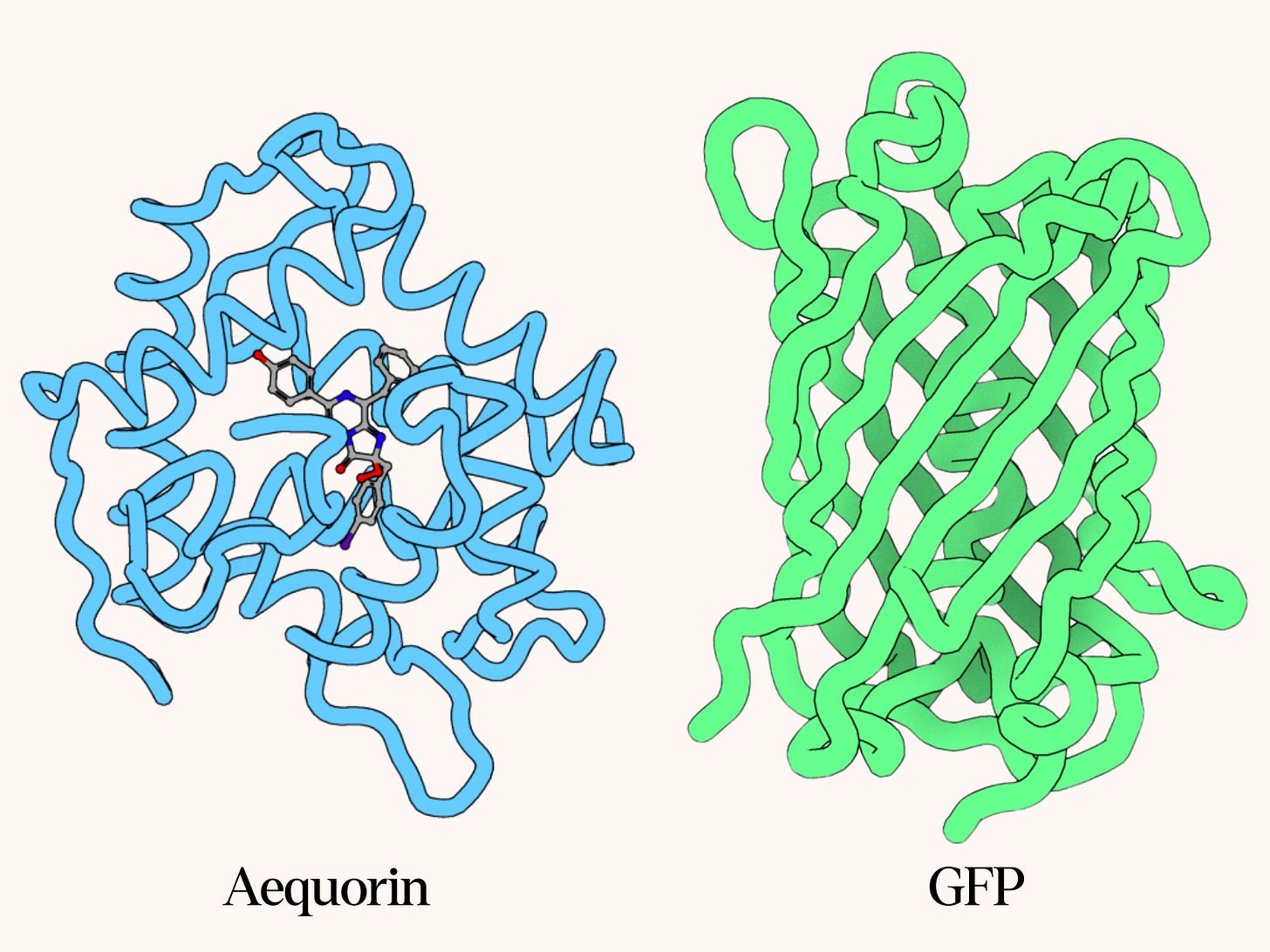
As aequorin’s utility grew, Shimomura felt increasingly compelled to understand its bioluminescent mechanism. After returning to Japan for a few years to study other bioluminescent species, he restarted his jellyfish work in Johnson’s lab in 1967.
Determining the structure and mechanism of aequorin demanded a gargantuan effort. Collecting enough aequorin for one structural study required about 50,000 jellyfish, and the team needed to conduct many studies over multiple seasons. Johnson’s ingenuity in crafting a jellyfish cutting machine, however, helped the team process up to 6,000 jellyfish per day. Johnson fashioned the device from reclaimed meat-slicing blades attached to a motor. Pressing the jellyfish against a slowly rotating spiked disk — a jellyfish “fork” — quickly separated thin strips from each animal’s outer ring.
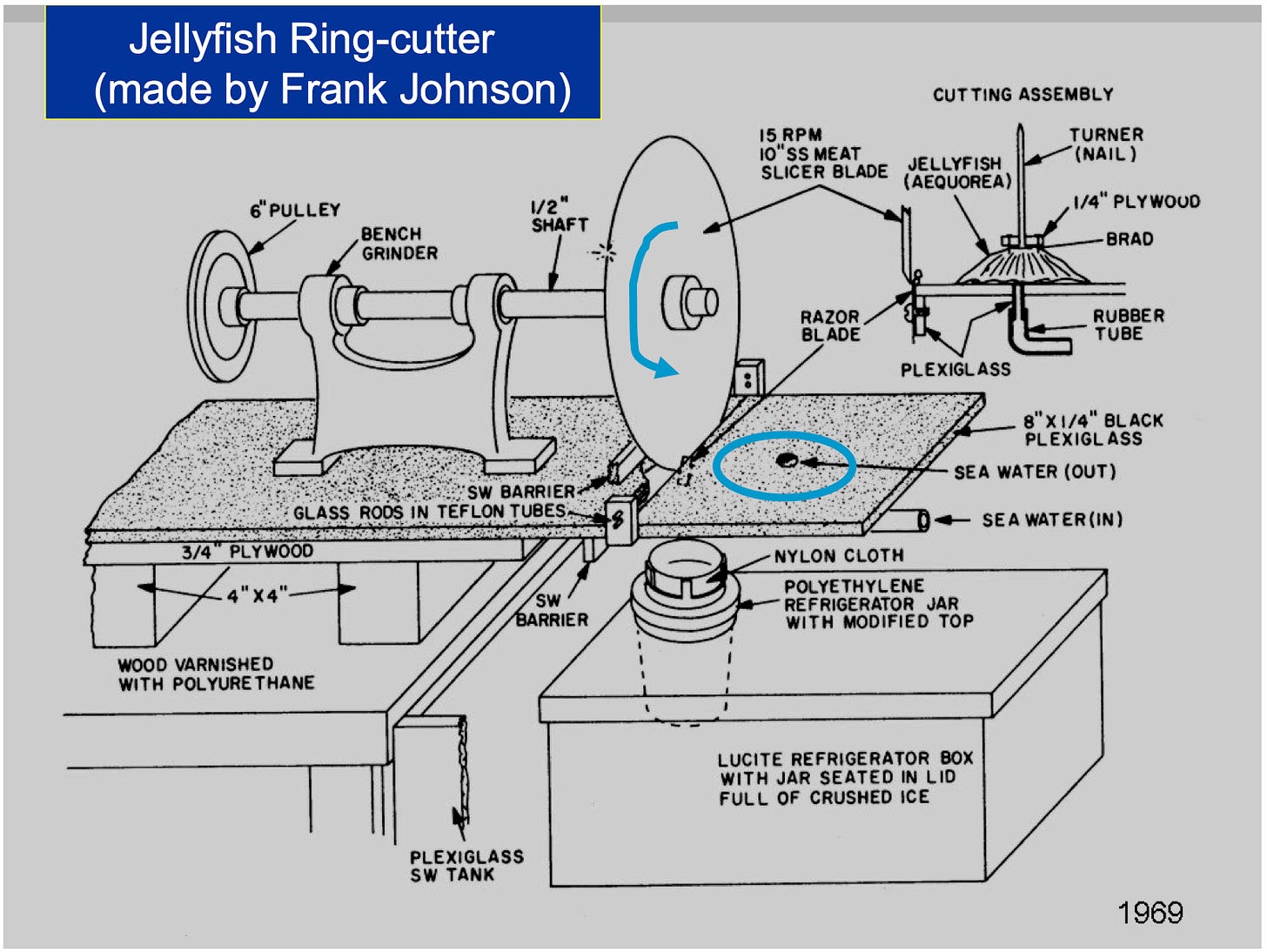
Shimomura initially focused on aequorin, but through that work, he laid the foundation for GFP’s far greater success. Since the light organs of Aequorea always contain both aequorin and GFP, Shimomura inevitably collected GFP alongside it. After five years of collecting jellyfish, he determined the structure of aequorin’s light-emitting component, publishing his findings in 1972. However, it took several more years to fully uncover the mechanism by which aequorin binds to calcium ions to produce blue light.
In 1974, Shimomura demonstrated that GFP absorbs aequorin’s blue light and then re-emits it as green, thus identifying the fluorescence mechanism and the jellyfish’s green glow. By 1979, he had collected enough purified GFP to analyze the amino acid sequence and piece together its structure, which turned out to be highly unusual. Whereas most fluorescent proteins require an external fluorescent molecule — called a chromophore — to function, GFP’s chromophore forms within its own amino acid chain. This self-contained fluorescence makes GFP stable and more suitable for cloning since it can be made from a single gene.
For the moment, GFP remained a niche protein. It carried an intriguing chromophore but otherwise had no immediate practical uses. Synthetic fluorescent dyes, such as fluorescein, provided similar absorption and emission characteristics and were simple to chemically synthesize; no need to cut up tens of thousands of jellyfish.
Summers at Friday Harbor became an annual tradition for the Shimomura family. Over two decades, he used the jellyfish for his own research and also supplied aequorin to colleagues. This was before DNA clones could produce these proteins synthetically, and few researchers were willing to endure the grueling work of collecting and cutting jellyfish. After 19 trips to Friday Harbor, the Shimomura family gathered more than 850,000 jellyfish.
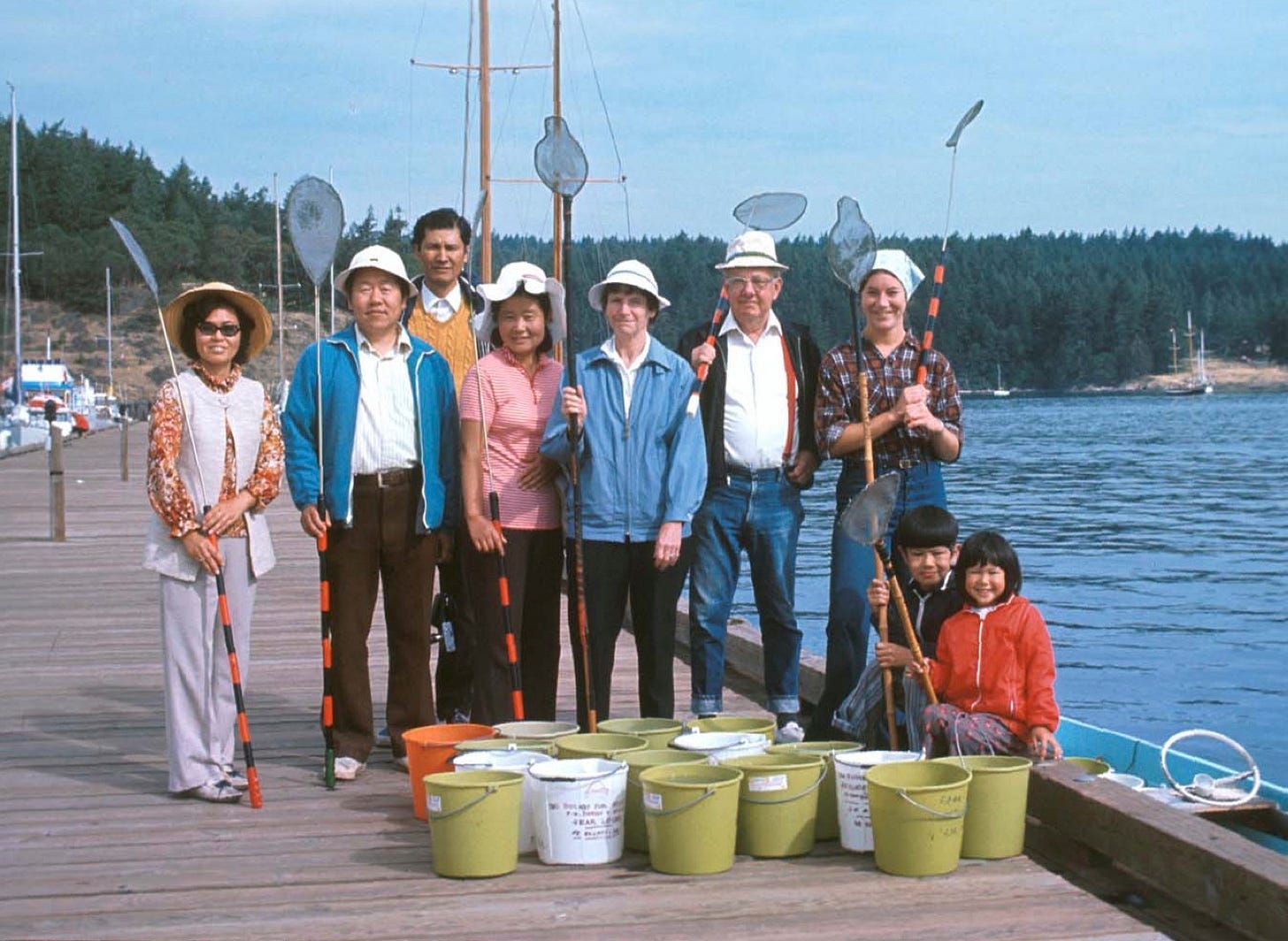
Although Shimomura and his team had become a GFP producing powerhouse, it was not where his true passion lay. He eventually returned to his broader interest in the basic science of bioluminescence, traveling the world to study it in different species: glow worms in the caves of New Zealand, luminous krill in Sweden, fireworms in Bermuda, and the red jellyfish Periphylla in the fjords of Norway. He later remarked that all these efforts were overshadowed by his role in GFP’s widespread adoption.
Shimomura had done his part for GFP. He had described its fluorescent nature and spectral properties, and then had determined its unique structure with a self-contained chromophore. But it was time for others with an eye towards applications to pick up the torch.
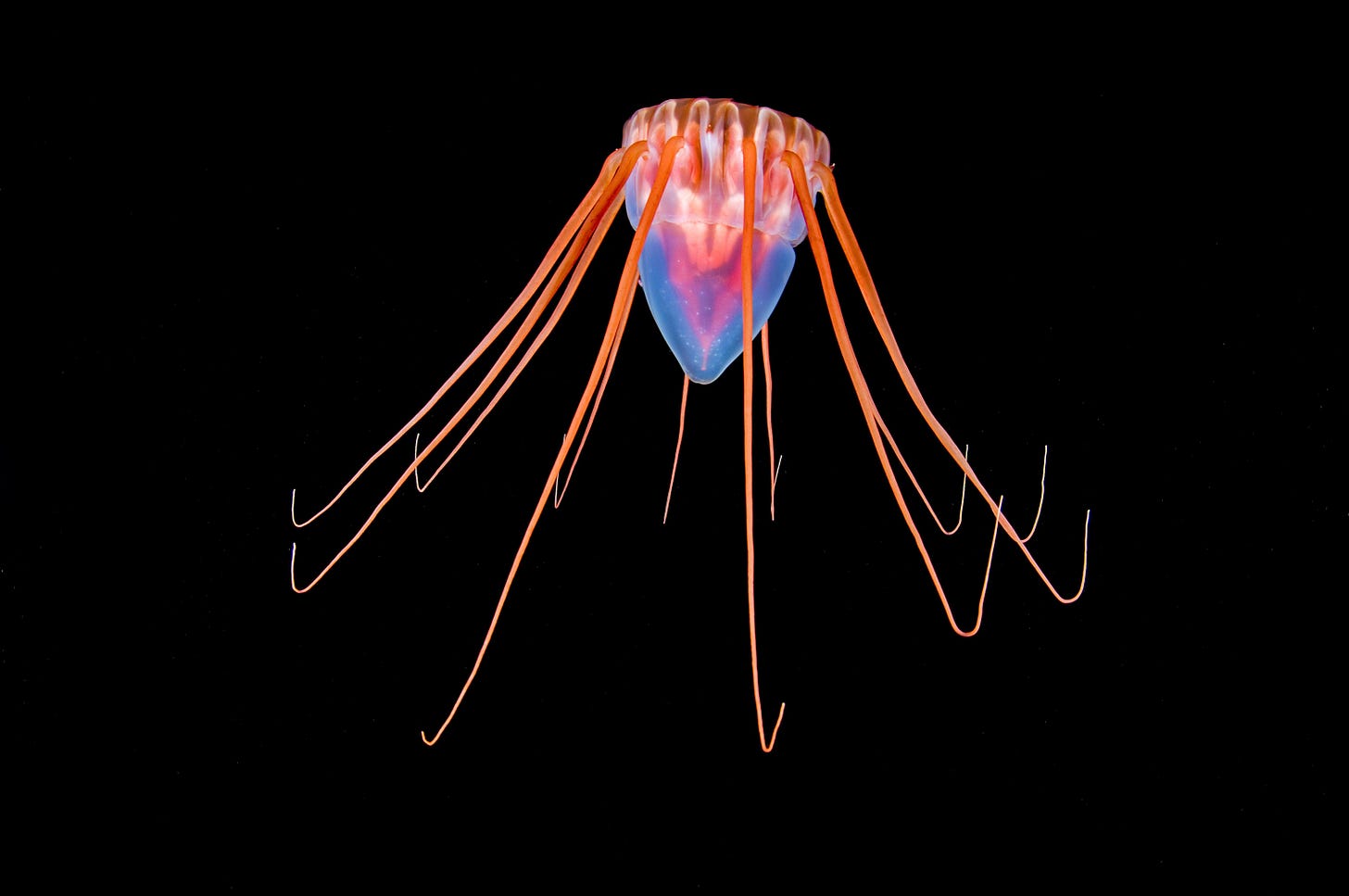
Passing the Torch
Douglas Prasher was a postdoctoral researcher at the University of Georgia in the 1980s when he began studying Aequorea. Like Shimomura, Prasher traveled to Friday Harbor to gather thousands of jellyfish and extract their light organs. But whereas Shimomura didn’t pursue practical applications for the glowing substance, Prasher suspected that it could be used to great effect in biology.
If a single gene could be expressed in any living organism to make this single-unit fluorescent protein, Prasher reasoned, then one could tag almost any gene or protein of interest and follow its progression inside a cell.
Prasher set out to find the GFP gene, and then clone it, by constructing a gene library from Aequorea jellyfish tissue. Each cell contains messenger RNA (mRNA) that carries instructions for making proteins, so Prasher first extracted mRNA from Aequorea and converted it into complementary DNA (cDNA). This cDNA, housed within a bacteriophage virus that easily replicates inside bacteria, holds copies of many jellyfish genes, including those for GFP. But Prasher needed a way to search through these genes and isolate the one responsible for GFP.
Fortunately, Shimomura and others had deduced part of the amino acid sequence for GFP several years before. Prasher used those sequences to design short, synthetic DNA probes that matched what he believed the corresponding DNA would look like. He then introduced these probes — radioactively labeled for easy detection — into the bacteria containing fragments of the jellyfish cDNA. Wherever a probe bound to the correct piece of DNA, the signal revealed a bacterial colony that possessed the GFP gene. By recovering the DNA from those colonies, Prasher could isolate the actual GFP gene and confirm its identity through further testing.
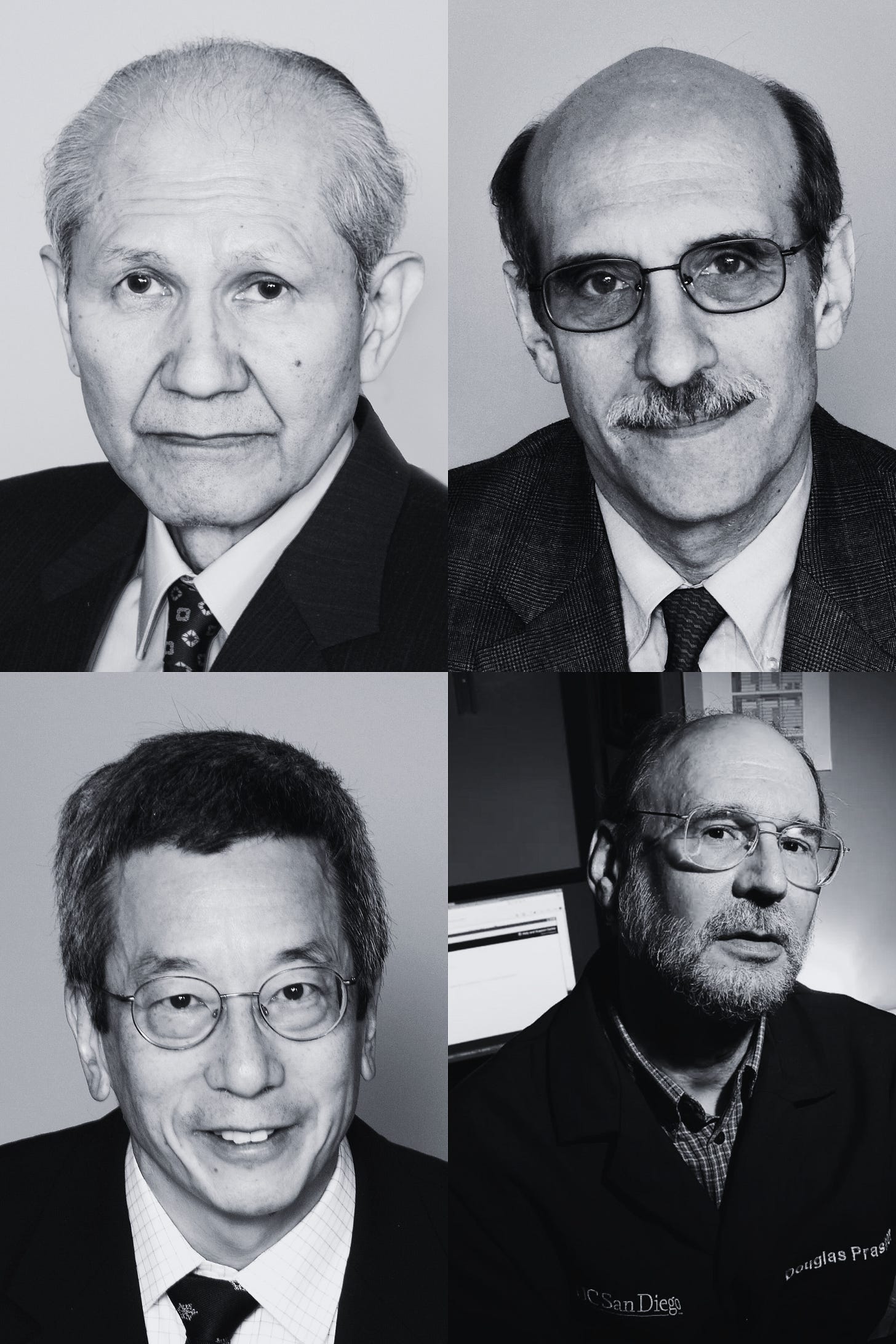
Once Prasher identified bacteria with the GFP gene, he used sequencing technology to determine its precise nucleotide sequence. At that time, most molecular biologists relied on the Sanger (dideoxy) chain-termination method for DNA sequencing. This process involved incorporating dideoxynucleotides — chemically modified nucleotides that halt further DNA extension — into a newly synthesized strand. The resulting fragments would then be separated by size on a polyacrylamide gel and visualized (usually via radioactive labeling), allowing for the deduction of the full nucleotide sequence one base at a time. Using this technology, Prasher successfully sequenced the GFP gene and opened the door to the protein’s use in other organisms.
By this time, Prasher had started his own lab in Woods Hole, Massachusetts, and sought to insert the GFP gene into various organisms — a crucial step in proving its usefulness as a cellular marker. But such a feat proved immensely challenging; at the time, scientists believed additional enzymes might be required to activate GFP’s fluorescence. When bacteria failed to glow, it was assumed that other jellyfish components were needed. Even Shimomura doubted whether GFP could ever function outside its native jellyfish environment.
Unfortunately, bioluminescence was deemed an esoteric field. Soon, Prasher’s funding ran dry, and he felt increasingly isolated as a molecular biologist surrounded by marine biologists at Woods Hole. However, before he abandoned GFP, he got two calls — one from Martin Chalfie and one from Roger Tsien. Both scientists had seen Prasher’s paper reporting the cloning of GFP DNA and asked if they could get a sample. Wanting to see GFP succeed, Prasher sent one to each.
Martin Chalfie, a neurobiologist studying touch sensation in the roundworm C. elegans, had become fascinated with GFP as a biological marker after learning about it at a seminar. When he received the GFP DNA from Prasher, he had two options: cut the DNA out with an enzyme, which would give extra non-coding jellyfish DNA, or use polymerase chain reaction (PCR) to amplify only the coding regions, a method that risked introducing mutations.
Chalfie chose to proceed via PCR. Within a month of receiving the DNA from Prasher, Chalfie succeeded in creating glowing bacteria and soon after that, glowing roundworms. The paper made the cover of Science in February 1994. In contrast, those who chose the enzyme method failed to get glowing bacteria, likely because the extra jellyfish DNA interfered, leading some to claim that additional jellyfish components were needed.
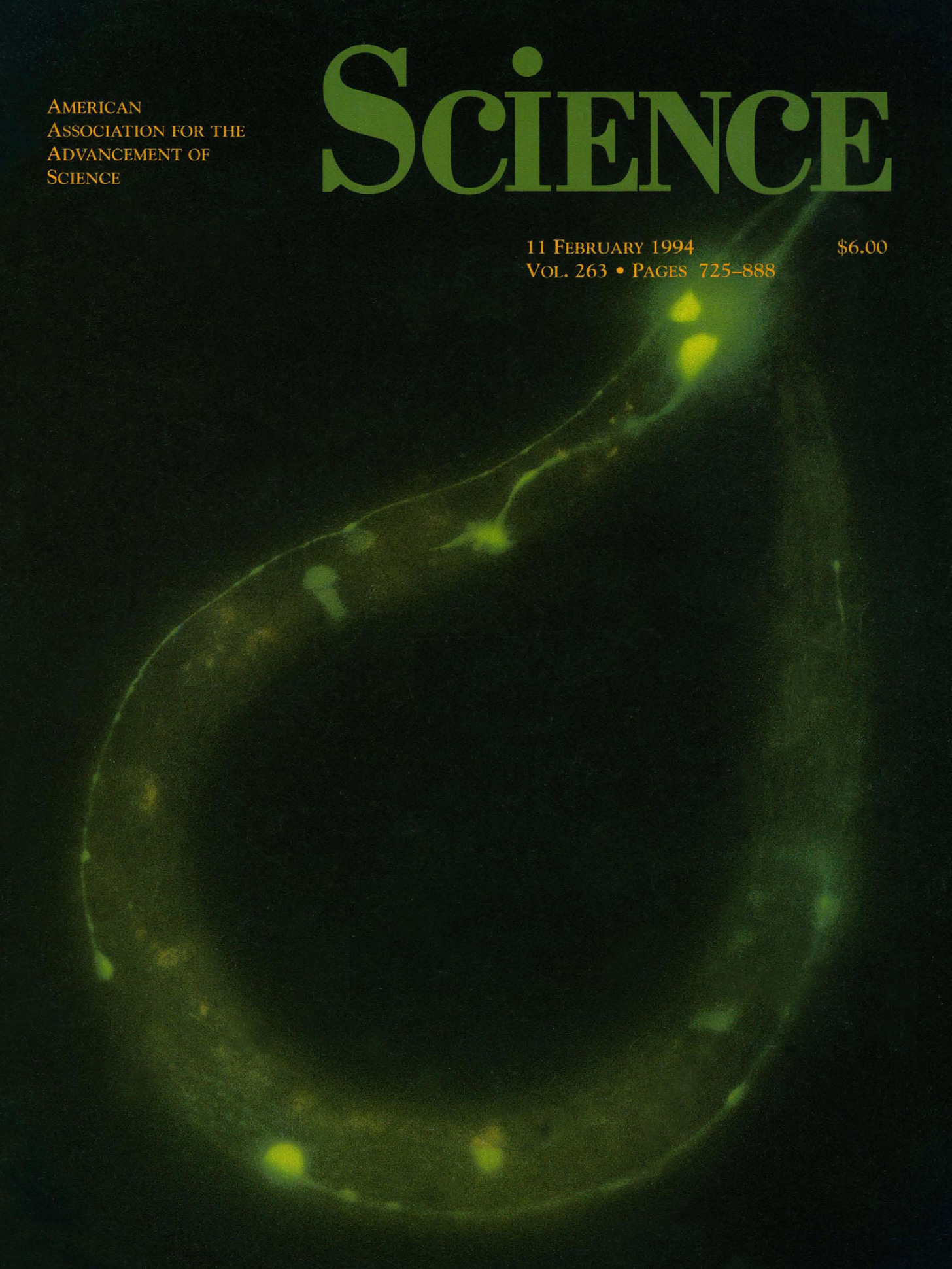
However, synthetic expression with “wild-type” GFP was not always reliable. For instance, when inserted into yeast, its fluorescence was very weak and highly variable across different cells. Tsien was determined to use his extensive background in developing fluorescent sensors to overcome this and show that GFP could become a revolutionary tool for visualizing biological processes.
Roger Tsien had been intrigued by chemistry from a young age. He grew up modifying household items to conduct chemistry experiments in his backyard and, on one occasion, even trying to synthesize aspirin. He studied neurobiology at Harvard and then swapped Cambridge, MA for Cambridge, UK for his PhD. While interested in neurobiology, he had become dissatisfied with traditional electrophysiology:
To me this seemed too much like ice fishing, i.e. cutting a hole in the ice covering a lake, dropping a fishing line into the opaque water beneath, and patiently waiting for a bite. The brain derives its power from trillions of neurons working in parallel, so I wanted to see lots of neurons simultaneously signaling to each other and processing information. Ideally one would stain the neurons with a dye that would visibly light up or change color whenever and wherever a neuron fired an action potential.
In response, he began developing better indicators for neuronal activity during his graduate and postdoctoral work. At the time, there were a few commercially available dyes that could respond to changes in membrane potential, but their optical responses were hard to detect. Tsien chose to work with calcium instead since neuronal action potentials cause larger and slower increases in calcium, making them easier to detect.
He was familiar with aequorin as a means of identifying calcium in cells. However, aequorin needed to be painstakingly injected into each cell in order to detect intracellular calcium activity. He also knew about GFP, having become interested in it when he learned that Prasher had cloned its DNA. Tsien chose to work with yeast instead of bacteria and began modifying the protein to improve its fluorescence.
Tsien’s work led to two significant contributions. First, he improved the fluorescence of wild-type GFP by introducing the S65T mutation. In its natural form, GFP absorbs both UV (395 nm) and blue (475 nm) light, making its fluorescence inconsistent and impractical for research. Tsien identified that Serine 65 (S65) played a key role in this dual absorption. By replacing it with Threonine (S65T), he stabilized the chromophore in a way that eliminated the UV absorption and shifted the excitation to a single, stronger absorption at 488 nm. This made GFP brighter, more stable, and ideal for fluorescence microscopy.
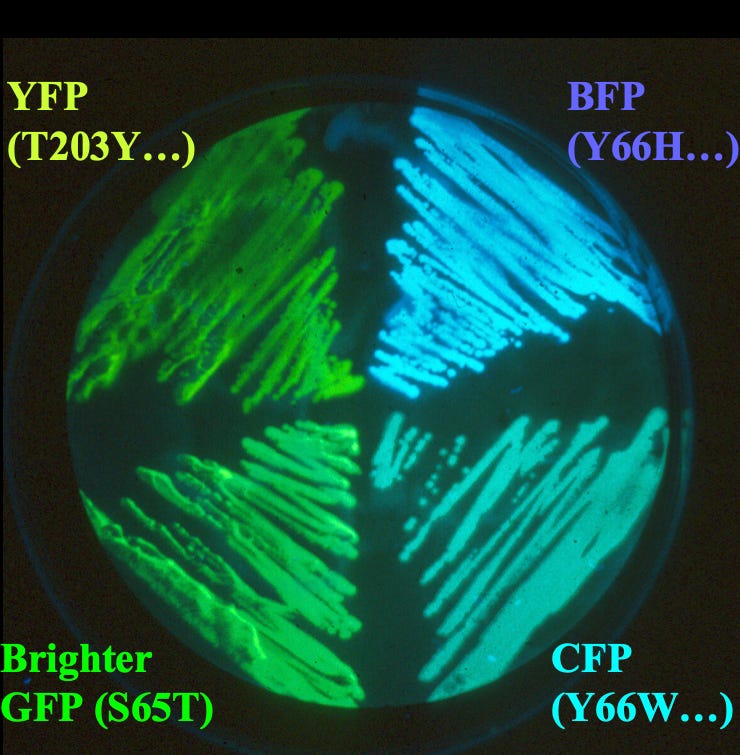
Second, Tsien’s research was primarily focused on co-fluctuations between two different cellular components. Tracking both of these components at the same time would therefore require at least two fluorescent colors. With his extensive training in creating synthetic dyes, then, Tsien decided to do precisely that; by randomly mutating the GFP gene to alter the chemical structure of wild-type GFP, he was able to create not just one other color but three: blue, cyan, and yellow fluorescent proteins. A mutation that introduced histidine at position 66, for example, created the blue fluorescent protein.
Further, a landmark 1999 paper reported the discovery of chromophores in non-bioluminescent corals, leading to the isolation of a red fluorescent protein called dsRed. Tsien modified dsRed to create a full spectrum of fluorescent proteins across the visible range. Since green and red wavelengths are more spectrally distinct than green and blue, using these two colors for visualization improved the accuracy of distinguishing signals from two sources. Today, dual-color imaging with GFP and RFP is a standard technique in biological research.
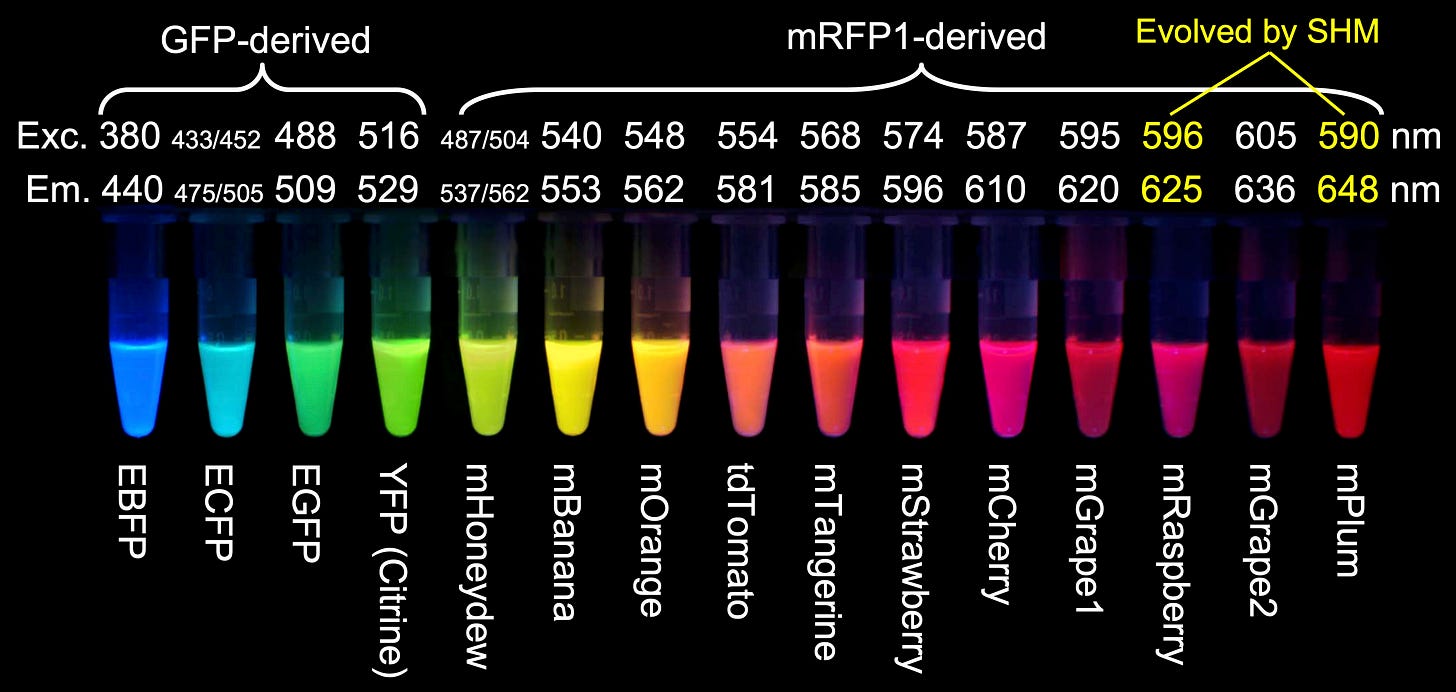
By enabling researchers to “light-up” living cells, GFP has played an indispensable role in neuroscience, cancer research, and even vaccine development. For example, in infectious disease and vaccine research, GFP has been crucial for studying how viruses spread within a host. In a 2015 study, researchers used GFP-tagged viruses to reveal HIV’s unique transmission patterns — an essential step toward developing therapies to prevent its spread. Or consider GFP’s role in neuroscience, where scientists are now able to capture the activity of over a million neurons simultaneously by engineering GFP into a glowing on-off switch.
From illuminating the frigid waters surrounding Shimumora’s rowboat to revealing molecular movements across synapses, GFP and its various colorful descendants have fundamentally changed how biological and physiological research is conducted. Shimomura, Prasher, Chalfie, Tsien, and countless others involved in GFP research have built upon what the jellyfish Aequorea victoria has been doing for more than 150 million years.
Listen to a behind-the-scenes interview with the author, Smrithi Sunil, on Spotify or Apple Podcasts.
Smrithi Sunil is a research scientist developing multiplexed and multimodal imaging technologies to study brain function. She received her PhD in Biomedical Engineering from Boston University.
Cite: Sunil, Smrithi. “A Brief History of GFP.” Asimov Press (2025). DOI: 10.62211/57yu-31kp
Lead image by Ella Watkins-Dulaney.
Interesting read!
Couple of minor points: luciferin and luciferase are classes of chemicals not individual chemicals and they are defined by their role in bioluminescence. So by definition the light emitting substance is luciferin and by definition the enzyme that drives it is luciferase. There's nothing to discover or prove at that level. The discoveries are what actual molecules are serving those functions in each species.
Secondly the New Zealand Waitomo caves are illuminated by fly larvae (altho the locals do call them glow worms).
For further reading I would recommend "Aglow in the Dark", a book from 2005 about the discovery and development of GFP. https://archive.org/details/aglowindarkrevol00vinc
Of course there's been a lot of progress since then, but the history is really interesting. (I will say that the authors of the book, both biologists, struggle somewhat with explaining the physics of fluorescence.)