Keeping Ahead of Contagion
We have the technology to detect airborne pathogens in real time. Now we must use it.
In October 2001, a quiet panic gripped the United States. Letters laced with anthrax spores began arriving at newsrooms and government offices, killing five and exposing thousands. Though the attacks were delivered through envelopes — not dispersed through the air — they exposed how everyday systems, like mail delivery, could become vectors for bioterrorism.
In the aftermath, the U.S. government launched BioWatch: an ambitious early-warning system meant to detect airborne biological threats in public spaces. Air-sampling units designed to collect and analyze air for traces of deadly pathogens like anthrax or smallpox were installed in more than 30 major cities. But the technology had a critical limitation. Samples first had to be collected on filters and then transported to laboratories for analysis — a process that took up to 36 hours. This approach could only catch an attack after the fact; it was too slow to prevent initial exposure or halt early transmission.
Since 2001, we’ve come a long way toward faster, more effective airborne disease detection. Enabled by breakthroughs like rapid genomic sequencing, portable PCR testing, and CRISPR-based diagnostics, technologies now exist that can detect pathogens in minutes rather than days. But to bridge the gap from proof of concept to commercial deployment, these devices still need to be made more resilient to fluctuations in temperature and humidity — and validated in realistic settings, from airports to hospitals.
Environmental instability poses serious challenges: high humidity can degrade reagents or cause condensation that interferes with electrical sensors, while extreme heat can denature the antibodies or enzymes used in many biosensors. Even dust and airborne contaminants can clog filters or cause signal drift, producing false readings or sensor failure. Most lab tests are conducted in controlled conditions — a far cry from the real-world environments like poultry barns or subway platforms in which they must operate.
As the bird flu spreads in the United States, the CDC should act with the same urgency it showed after the 2001 anthrax attacks, when it deployed the Laboratory Response Network and launched the BioWatch program. If we can clear these remaining hurdles, real-time pathogen monitoring could become commonplace in just a few years, transforming outbreak response from reactive damage control into proactive prevention.
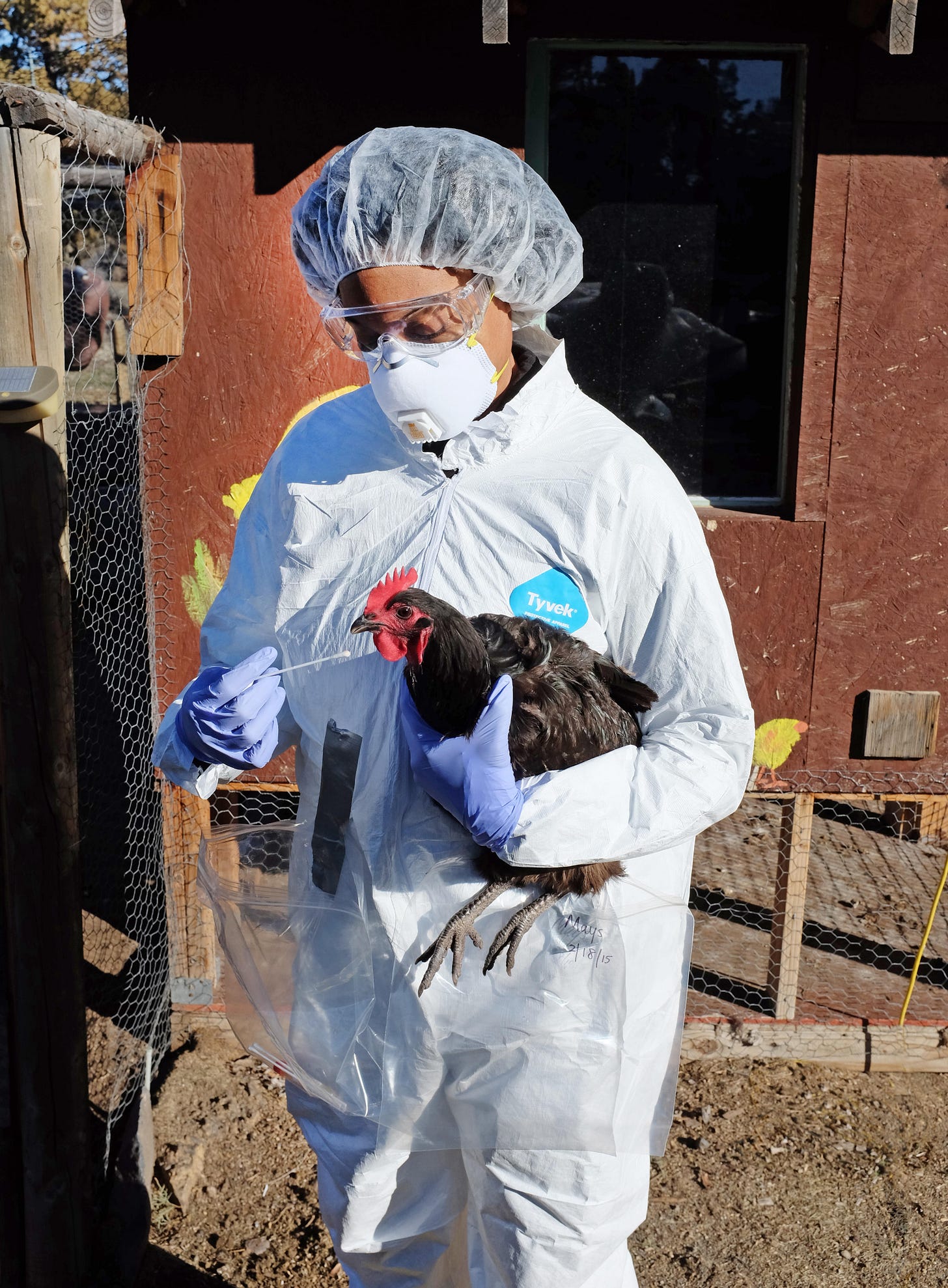
The Speed Advantage
Time is biology's great multiplier. When an infectious disease goes unchecked, each case multiplies exponentially. If one infected person spreads a disease to two others (R₀= 2), 64 cases will appear within 30 days — all of which could have been prevented by detecting and halting that first infection.
The 2014-2016 Ebola outbreak in West Africa, for example, began quietly in a Guinean village and went unrecognized for months. By the time health officials confirmed Ebola, which has an average R₀ of 1.95, as the culprit, the virus had already spilled into neighboring countries. More than 11,000 people died over the course of the outbreak. According to modeling studies by the WHO Ebola Response Team, published in the New England Journal of Medicine, earlier identification and intervention in late 2013 could have dramatically reduced transmission — potentially sparing thousands of lives.
One reason that Ebola was able to spread undetected for so long is that it began in a remote area with weak health surveillance, and its early symptoms resemble common illnesses like malaria and typhoid — leading to misdiagnosis and delayed reporting.
But even when surveillance systems are in place, the effectiveness of outbreak response often hinges on the speed and accuracy of diagnostic tests. Testing strategies are defined by a fundamental trade-off: faster tests tend to be less accurate, while highly accurate tests take longer to process. Currently, we have two primary options for detecting infectious diseases: rapid antigen tests and laboratory-based molecular methods. Each has distinct strengths and weaknesses.
Rapid antigen tests detect viral surface proteins — physical fragments of the virus, like the spike or nucleocapsid proteins — using antibodies embedded in a test strip. When a nasal swab sample is applied, the viral proteins (if present) bind to those antibodies and produce a visible signal, typically within 10 to 30 minutes. These tests are fast, inexpensive, and easy to use outside of lab settings. But their sensitivity varies widely, from 34 to 80 percent, especially in the early stages of infection. That’s because the virus may not have produced enough protein to trigger a detectable response.
PCR tests, by contrast, detect the virus’s genetic material, its RNA, rather than its surface proteins. After a sample is collected, usually via swab, it’s sent to a lab where the viral RNA is extracted, converted into DNA, and placed into a thermal cycler — a machine that heats and cools the sample repeatedly to trigger a chain reaction that amplifies the genetic material. Even a tiny amount of viral RNA can be multiplied millions of times, making PCR far more sensitive than antigen tests (typically 93 to 98 percent). This amplification process takes only a couple of hours. But because PCR requires specialized equipment, trained technicians, and controlled lab conditions, the full testing workflow — including sample transport, batching, and lab backlogs — can stretch total turnaround time to 24 to 48 hours. In fast-moving outbreaks, such delays can mean the difference between containing a virus and letting it spread.
Finding the right balance between speed and accuracy often boils down to context, particularly how quickly the disease spreads, how severe the outcomes are, and the cost of false results. The steeper a pathogen’s reproduction rate (R₀), the more crucial it becomes to detect new cases as early as possible. Norovirus outbreaks, which can have an R₀ of 2-3, are a prime example: waiting even 24 hours for a near-perfect result can mean missing the window to isolate sick individuals or close contaminated spaces, causing dozens of secondary infections. The disease is known for its swift and explosive outbreaks on cruise ships or in dormitories and routinely features cases where a single individual infects dozens of others within 48 hours. In the case of a recent outbreak on a cruise ship bound for the Caribbean, 224 passengers and 17 crew members out of 2,538 total onboard were affected within 10 days.
Diseases resulting in worse outcomes, like meningococcal meningitis — which can progress from mild flu-like symptoms to severe sepsis, brain swelling, and death within 24 hours if untreated — require even speedier detection. This rapid detection is most useful when positive results trigger relatively mild protocols, like temporary isolation or a short-term antiviral for the flu, rather than more invasive treatments.
The setting also matters: in a hospital dealing with immunocompromised patients, even a single missed infection can be catastrophic. For a cancer patient undergoing chemotherapy or a newborn in intensive care, exposure to a virus like RSV or norovirus can lead to life-threatening illness — far more severe than in a healthy adult. In these settings, false negatives can prove fatal and make accuracy all the more important.
On the other hand, in a large-scale community outbreak, the aggregate benefit of speed tends to override the detriments of some false negatives since quick action can avert hundreds of subsequent infections. Research shows that, across infectious diseases, only 20 percent of cases generally cause about 80 percent of transmission. This makes early detection of these "super-spreader" events especially valuable: stopping just one of these cases early can prevent dozens or even hundreds of downstream infections. For example, during the 2015 MERS outbreak in South Korea, a single patient was ultimately linked to over 80 subsequent infections in the Samsung Medical Center.
When faced with a potential outbreak, researchers model trade-offs through “testing efficacy,” which measures how different combinations of speed, sensitivity, and tracing efforts impact overall transmission. In a 2021 study, researchers simulated scenarios where a slower, more accurate test was compared to a rapid test with higher false negatives in the context of COVID-19. They found that even if a fast test missed up to 40 percent of infections, it could still curb the virus’s spread more effectively than a slower but near-perfect test, largely because every extra day (or hour) of waiting gives a contagious individual time to infect others. A test delivering results in a few hours with 60 percent sensitivity would perform comparably to a test delivering results after 3 days with 100 percent accuracy. In other words, speed matters more than accuracy for breaking chains of transmission for most diseases, although settings with vulnerable populations or poor tracing capacity may still require highly sensitive, slower testing.
But, often, we need to test people before they have any reason to seek testing at all. This is especially true for diseases for which presymptomatic transmission is common, like influenza, rather than those where transmissibility peaks after symptom onset, like Ebola. For example, 44 percent of COVID-19 transmissions occurred before symptoms developed. Accordingly, a Harvard School of Public Health model shows that frequent rapid testing every three days, including among asymptomatic individuals, could reduce transmission by 88 percent, even with tests that missed 30 percent of cases.
Early Sampling
One approach to achieving this would be to preempt infection. The idea of sampling air to detect disease dates back nearly a century, but gained traction in the early 20th century during efforts to control tuberculosis and other respiratory illnesses. In the 1930s and 40s, scientists developed the first purpose-built air samplers — mechanical devices that pulled air through narrow inlets to capture particles on filters or petri dishes. One of the earliest widely used models was the Andersen air sampler, introduced in the 1950s by engineer Sam Y. Andersen at the U.S. Public Health Service.
The Anderson device worked by pulling air through a series of stacked metal plates, each with holes of different sizes. As the air flowed through the device, heavier and larger particles were forced out of the airstream first and landed on the upper plates, while smaller particles penetrated further down. Each plate held a petri dish containing a layer of nutrient-rich agar, where microbes in the particles would grow into visible colonies over time. This multi-stage design let researchers not only detect which microbes were present but also estimate how deep into the lungs those particles could travel. Larger particles would get trapped in the nose or throat, while smaller ones would reach the lungs or even bloodstream.
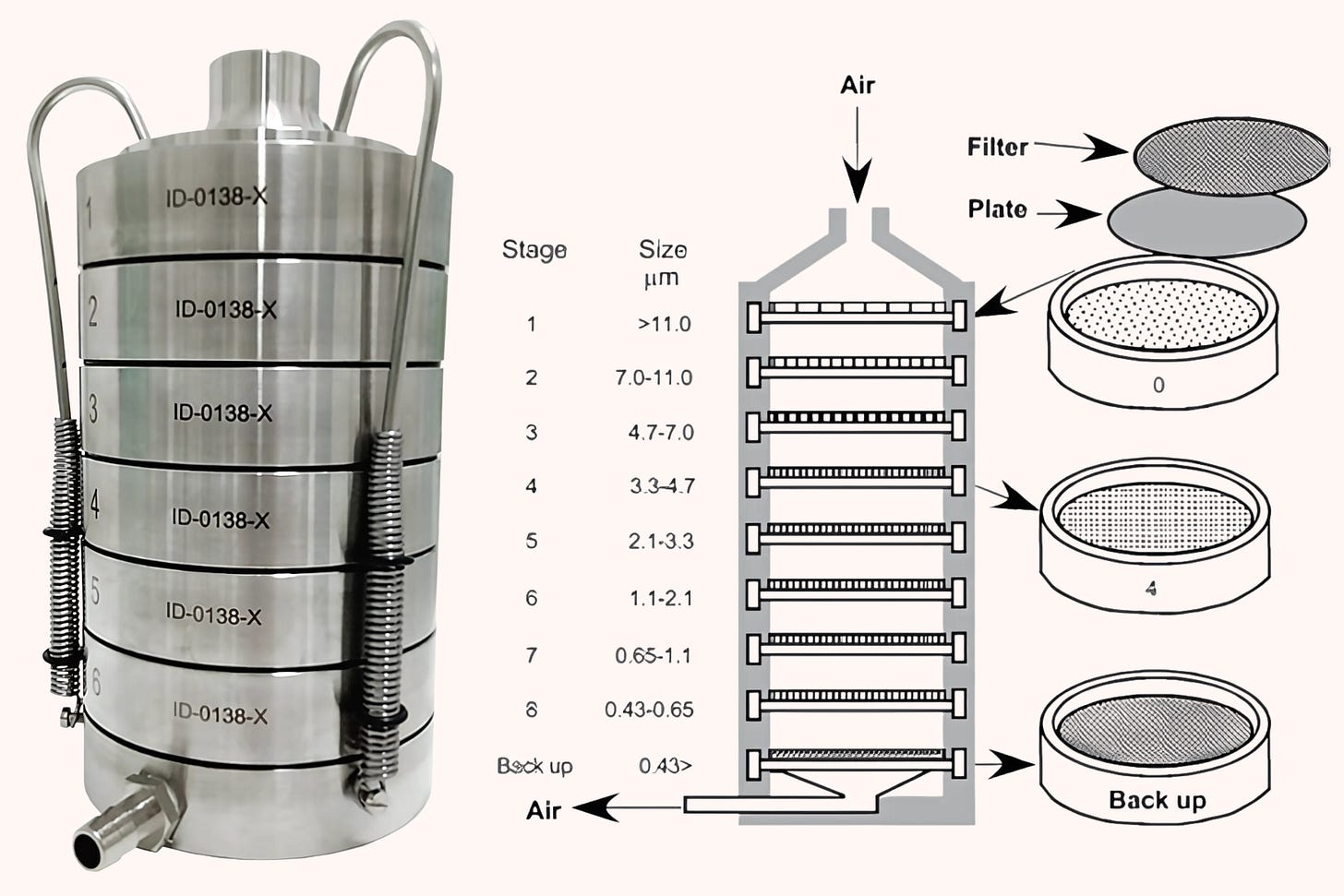
The process was slow and analog. Once samples were collected, researchers would incubate the agar plates for up to 48 hours and wait to see what, if anything, grew. This method could identify common pathogens like Staphylococcus aureus or Mycobacterium tuberculosis, but it had major limitations: it required viable (still-living) organisms, couldn’t detect viruses, and produced results far too slowly to inform real-time outbreak response. Still, these samplers were a breakthrough in understanding the airborne transmission of disease and formed the backbone of early infection control in hospitals, operating rooms, and military settings.
By the 1960s and 70s, scientists developed more compact and specialized air samplers. Slit-to-agar samplers drew in air and funneled it through a narrow slit, allowing particles to land directly on a rotating agar plate for culturing. Liquid impingers bubbled air through a liquid medium that trapped airborne microbes, which could then be analyzed in a lab. And portable filtration units used small pumps to pull air through fine filters that captured dust, bacteria, and spores, an approach that made it easier to test for airborne pathogens in field settings like farms, schools, or crowded buildings. While none could offer real-time detection — most still relied on culturing microbes after collection, meaning researchers had to wait 24 to 72 hours to see if anything grew on the agar plates or in the liquid media — they laid the foundation for the idea that airborne pathogens could be systematically captured and studied. These early devices also made it clear that the air we breathe is rarely sterile.
Bioterrorism threats like anthrax spores and emerging global outbreaks such as SARS ignited interest in new tools capable of detecting pathogens in real or near-real time in the early 2000s. Government agencies and academic laboratories began seeking faster, more portable detection methods. In 2003, the U.S. government launched the BioWatch program — the country’s first large-scale attempt at continuous environmental biosurveillance. The system placed air-sampling units in over 30 major cities, often atop buildings or transit hubs, to detect airborne bioterror agents like anthrax or tularemia. These devices used filters to passively collect particles from the air over 24-hour periods, which were then manually retrieved each day by technicians and sent to public health laboratories. There, samples underwent PCR testing to identify the DNA of specific pathogens.
While innovative for its time, BioWatch was far from real-time: the full cycle, from air collection to lab analysis, took 12 to 36 hours, making it too slow to stop exposure in the event of an outbreak. Its reliance on preset detection targets and fixed locations also left blind spots in both time and geography. Despite these limitations, BioWatch represented a shift toward proactive disease monitoring and set the stage for later innovations in rapid, on-site detection.
More compact molecular testing units also began appearing in specialized settings like hospitals, mailrooms, and border checkpoints. One of the most prominent was Cepheid's GeneXpert platform, a self-contained PCR machine about the size of a desktop printer. While not lightweight — a standard unit weighs around 60 pounds — GeneXpert dramatically reduced the footprint of traditional PCR testing, which typically requires multiple lab instruments, cold storage, and trained technicians working in controlled environments. Instead, GeneXpert uses sealed, disposable cartridges and automated processing: a user inserts a clinical sample, and the machine performs RNA extraction, amplification, and detection internally, delivering results in under two hours. Staff had to actively operate the air sensors, but they brought fast, accurate molecular testing directly to the point of care.
In one 2011 multi-site U.S. study, GeneXpert showed 98 percent sensitivity in smear-positive tuberculosis cases and 72 percent sensitivity in smear-negative ones, with less than five minutes of hands-on time and a total turnaround of under two hours. While still reliant on electricity and indoor use, GeneXpert systems helped bridge the gap between centralized lab testing and rapid field diagnostics.
Advanced Surveillance
Today, four main types of airborne pathogen detectors are in the works, each with distinct trade-offs in sensitivity, speed, scalability, and real-world applicability.
Optical sensors detect airborne particles by shining a beam of light, usually from a laser or LED, through the air. As particles pass through the beam, they bend, block, or reflect the light in different ways. Detectors then capture these changes and analyze them to estimate the particles’ size, shape, and sometimes surface texture. Some advanced systems, like a 2022 prototype from UCLA, take this a step further by creating holographic images of each particle and using artificial intelligence to try to identify whether it might be a pathogen.
But while optical sensors are fast — delivering results in under a second — they face major limitations. They can’t identify genetic or protein-level features, so they struggle to distinguish between harmful pathogens and benign particles of similar size and shape, like pollen or dust. Their detection threshold is also size-dependent: most can’t reliably pick up particles smaller than 0.5 microns, which excludes many viruses. Their sensitivity and specificity vary widely depending on the environment and pathogen, but most optical systems are not considered diagnostic-grade — meaning they can't reliably confirm whether a specific pathogen is present or absent with the level of accuracy needed to guide clinical or public health decisions.
Costs typically range from $10,000 to $50,000 per unit, and while they require little sample processing, they still need frequent calibration and can be affected by humidity or ambient light. These trade-offs make optical sensors better suited for preliminary screening or environmental monitoring, like detecting shifts in air quality in airports or HVAC systems, rather than definitive pathogen identification.
Mass spectrometry-based devices detect pathogens by analyzing the chemical makeup of airborne particles. First, air is drawn into the machine and particles are collected, typically using filters or impactors. These particles are then ionized, meaning they're broken into charged molecules. The machine measures the mass and charge of each fragment, producing a chemical “fingerprint” that can be compared to a database to identify the presence of a pathogen. This method doesn’t rely on genetic material or antibodies, making it especially useful for identifying a broad range of known — and potentially unknown — pathogens, along with features like antibiotic resistance or toxin profiles.
The main strength of mass spectrometry is its versatility and precision: it can detect multiple pathogens, distinguish between closely related strains, and even flag chemical markers of emerging variants. But it’s also among the least practical for real-time surveillance. Instruments are expensive — typically $100,000 to $500,000 — and require trained operators and controlled lab conditions. Sensitivity and specificity vary by protocol and setup, but recent studies have shown performance on par with PCR for certain pathogens.
In 2023, researchers at the University of Pavia in Italy developed a mass spectrometry-based breath analyzer that could detect SARS-CoV-2 with sensitivity and specificity both above 90 percent, analyzing samples in just 5 minutes. However, the system still relied on careful sample prep and manual handling. It also may be more difficult to differentiate and characterize individual components because the device pulls in many unique molecules.
Overall, mass spectrometry offers powerful analytical capabilities but remains best suited to research or high-resource clinical settings. These systems are too costly and complex for routine deployment in places like schools, farms, or airports, at least for now.
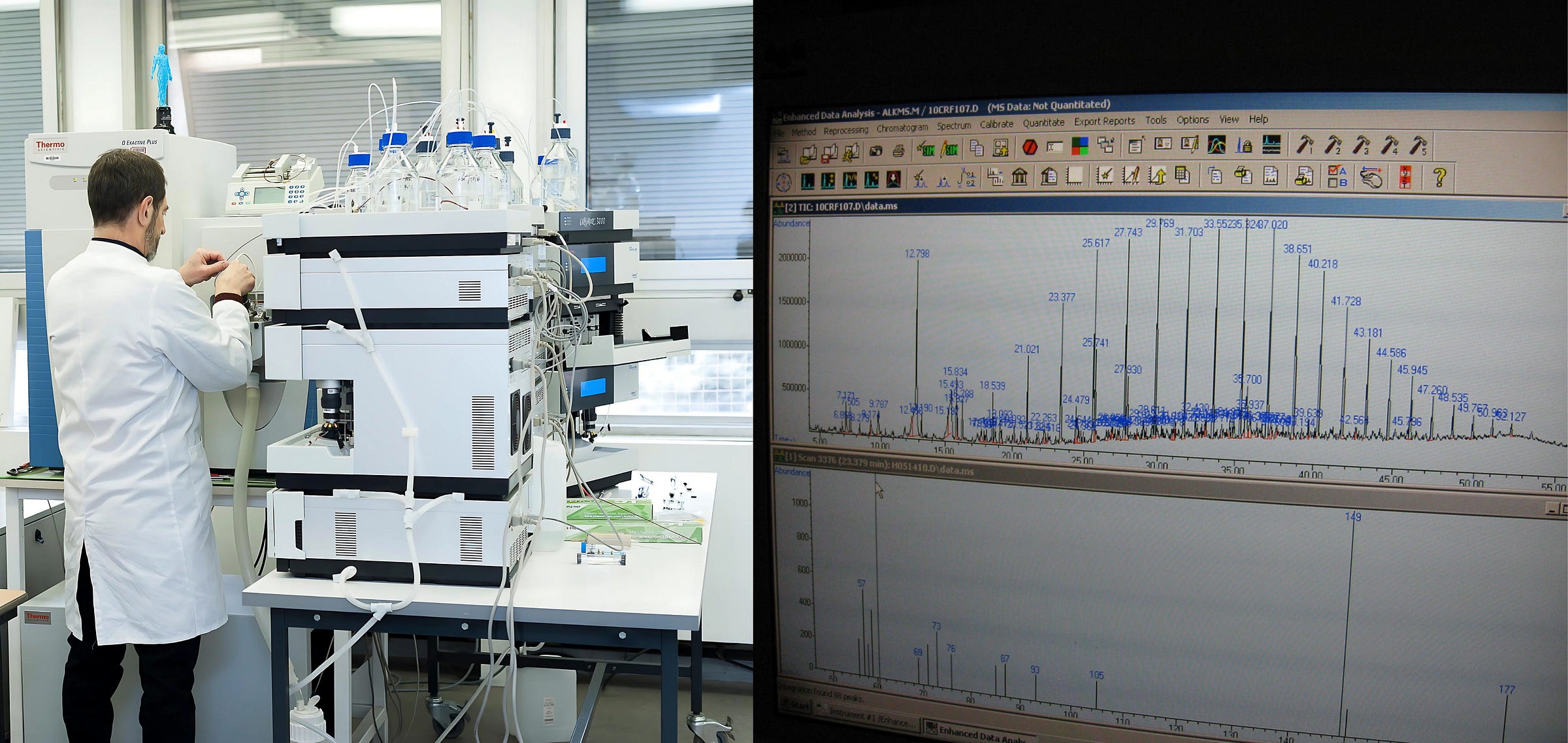
Nucleic acid amplification systems detect pathogens by identifying and copying tiny amounts of their genetic material, either DNA or RNA, until there’s enough to measure reliably. The most common method is PCR, which uses cycles of heating and cooling to replicate viral or bacterial genes millions of times. This amplification makes PCR extremely sensitive: even a few strands of viral RNA in a sample can be enough to trigger a positive result.
Although highly accurate, with sensitivities often above 95 percent and specificities near 100 percent, PCR requires stable temperatures, specialized enzymes, and trained technicians, which limits its use outside of controlled laboratory settings. Commercial PCR machines cost between $3,000 and $30,000, and processing times range from 2 to 6 hours, not including transport and batching delays.
To overcome these constraints, researchers have developed faster and more portable alternatives. Loop-mediated isothermal amplification (LAMP) performs genetic amplification at a constant temperature, eliminating the need for thermal cyclers and allowing for simpler devices. CRISPR-based diagnostics use guide RNAs to recognize target genetic sequences and trigger a detectable signal, often within 30 minutes. These newer methods can deliver nearly PCR-level sensitivity with fewer technical requirements.
But real-world validation of these newer systems is still limited. In 2022, researchers at Jiaxing University in China deployed a CRISPR-based biosensor in hospital ICUs to detect airborne E. coli. The system combined robotic air sampling with nucleic acid extraction and CRISPR amplification, delivering results in under an hour. However, most of these platforms remain in early stages, with few outside pilot settings. Like traditional PCR, they’re also sensitive to heat, humidity, and dust — all of which can degrade nucleic acids or interfere with reagents.
Micro-immuno electrodes detect airborne pathogens by using tiny, antibody-coated sensors that bind to virus particles in the air. When a pathogen, like the spike protein of SARS-CoV-2 or hemagglutinin from H5N1, makes contact with the antibody, it causes a measurable change in electrical current. This electrochemical signal is picked up almost instantly, producing a result in under five minutes. MIEs don’t require gene amplification or complex optics; they detect the physical presence of a virus in real time, directly from the air.
These devices are small, fast, and inexpensive to produce. Prototypes range from $500 to $5,000, with per-test costs as low as a few dollars. Because MIEs don’t need reagents, cold storage, or trained lab personnel, they are far more deployable than most other technologies.
Compared to other airborne detection methods, MIEs are the most promising for near-term commercial use. Optical sensors are fast but can’t reliably detect smaller particles or distinguish between viruses and dust. Mass spectrometry offers high precision but is too expensive and requires specialized operators. Nucleic acid amplification (like PCR or CRISPR) remains the gold standard for accuracy but is slower, more expensive, and still largely lab-bound. MIEs, by contrast, are affordable, fast, and capable of autonomous operation without major infrastructure — a combination that makes them uniquely attractive for real-time monitoring in airports, hospitals, farms, and more.
Still, MIEs aren’t perfect. Their biggest technical hurdle is environmental sensitivity: factors like heat, humidity, and airborne dust can interfere with the biosensor’s performance — a problem known as “biofouling.” For instance, high humidity can change the conductivity of the sensor’s surface, while dust and organic material can block antibody binding sites. According to a 2023 review in Biosensors and Bioelectronics, improving surface coatings and filtration methods could mitigate these effects without overhauling the core design. Unlike PCR (which depends on cold-chain enzymes) or mass spectrometry (which relies on clean air inputs), MIE limitations stem from the deployment environment rather than fundamental drawbacks in the method — making them more tractable to solve with better engineering and materials.
From Lab to Field
In February 2025, researchers at Washington University published a paper detailing a new H5N1 sensor — a concrete example of MIE technology in action. The device rapidly collects airborne particles through a "wet cyclone," trapping viruses in a specialized liquid solution. This solution flows past electrodes coated with antibodies specifically designed to bind to the H5N1 virus. When a virus particle binds, it triggers a signal within minutes, confirming its presence. Initial prototypes cost around $10,000 each to build, but the researchers anticipate these costs dropping below $1,000 per unit at scale, with each test costing less than a dollar.
This technology could be commercialized within the next two to three years if funding and validation trials proceed as planned, on the assumption that these devices — based on the team’s current knowledge — don’t appear to require either FDA or EPA approval. If that’s true, a wider roll-out of real-time airborne pathogen detection may be possible as soon as 2030.
To get there, the CDC should establish targeted public-private partnerships to support funding and scaling of promising biosensor technologies. One clear model is the NIH’s Rapid Acceleration of Diagnostics (RADx) initiative, which fast-tracked COVID-19 test development through a mix of government funding and private-sector partnerships. A similar effort, let’s call it “RADx-Air”, could be launched specifically for airborne pathogen detection, leveraging funding from BARDA (Biomedical Advanced Research and Development Authority) and operational support from the USDA to pilot sensors in high-risk agricultural settings.
For commercialization, partnerships with biotech firms like Cepheid (which specializes in rapid molecular diagnostics), Abbott (developer of the BinaxNOW test), or Nanōmix (which has experience with portable biosensors) could accelerate mass production. Meanwhile, agricultural leaders like Tyson Foods and Perdue Farms could serve as early adopters, testing sensors in poultry and cattle operations. Smaller-scale livestock producers, who often lack access to real-time surveillance tools, could particularly benefit from low-cost, easy-to-use biosensors deployed at milking parlors, ventilation systems, and transport hubs.
Beyond industry partnerships, Congressional backing could fast-track the deployment of airborne biosensors through dedicated funding. A federal biosurveillance initiative, modeled after the CDC’s wastewater surveillance program for COVID-19, could provide real-time data on emerging airborne threats. A White House directive, similar to Operation Warp Speed, could spur investment and coordination across agencies.
The CDC should also develop standardized validation protocols for field-testing these new technologies in realistic environments — similar to the roll-out of rapid PCR testing under the CDC-led Laboratory Response Network, after the 2001 anthrax attacks.
Despite promising technical progress, the biggest bottleneck may be institutional inertia. State and federal policymakers, CDC officials, and agricultural leaders should push for immediate funding to move from prototype to field deployment. In today’s political climate, a sweeping federal initiative like Operation Warp Speed is unlikely. But airborne biosensors aren’t just pandemic-prevention tools — they could help protect a cornerstone of the U.S. economy.
The current outbreak of H5N1 has already jumped from poultry to dairy cattle, raising the risk of widespread economic fallout. In 2015, the worst avian influenza outbreak in U.S. history ripped through the poultry industry, infecting over 50 million birds across 15 states. The virus spread so rapidly that containment was impossible — farmers were left with no option but to cull entire flocks at staggering economic and ethical costs. The outbreak wiped out 12 percent of the country’s egg-laying hens, causing egg prices to skyrocket by 61 percent relative to the previous year and poultry export bans that cost the industry an estimated $3.3 billion. Despite these losses, response efforts were largely reactive: by the time infections were confirmed, it was already too late to prevent mass destruction. Rather than waiting for human transmission to surge, public investment in early detection could form an agricultural defense.
Now, a decade later, we face another mounting outbreak, but we are far better equipped scientifically than we were in 2015. Imagine if airborne pathogen sensors had been deployed in poultry barns back then. Real-time detection of H5N1 could have alerted farmers to viral presence before birds fell sick, allowing for targeted interventions rather than indiscriminate culling. Instead of scrambling to contain an outbreak after the fact, producers could have acted immediately — isolating affected areas, adjusting biosecurity measures, and preventing catastrophic spread, all while saving billions of dollars.
This is not a question of feasibility but of political will. The technology exists. The 2015 outbreak should have been a wake-up call. Today, with real investment in airborne pathogen monitoring, we could avert another crisis if we embrace these tools in time.
Deena Mousa, a Works in Progress + Asimov Press Writing Fellow, is also a researcher, grantmaker, and journalist focused on global health, development, and scientific and technological progress.
Cite: Mousa, D. “Keeping Ahead of Contagion.” Asimov Press (2025). https://doi.org/10.62211/21kj-88qw
Lead image by Ella Watkins-Dulaney.